Short Circuit: The High Cost of Electric Vehicle Subsidies
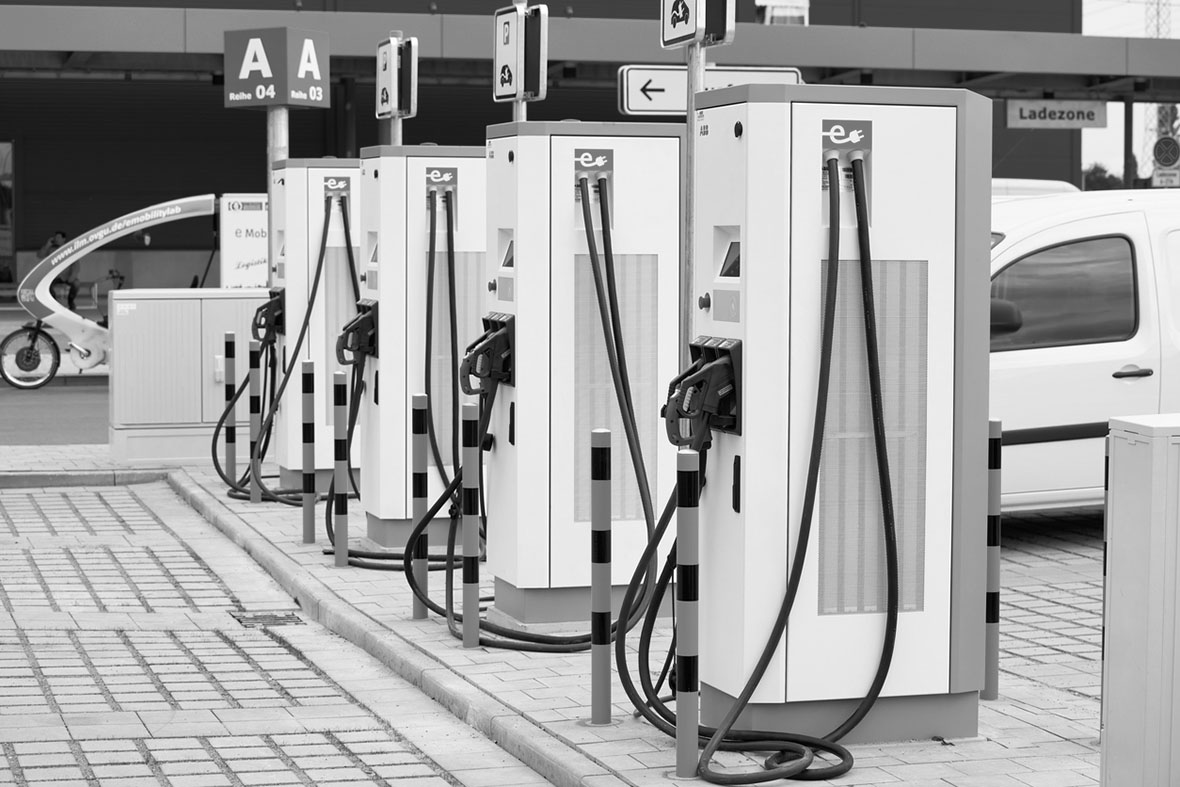
Many claim that “zero-emissions vehicles” (ZEVs), especially battery-powered electric vehicles, should replace most, if not all, cars and trucks powered by gasoline-burning internal combustion engines. The primary rationale is to reduce air pollution and carbon dioxide (CO2) emissions.
To effect this change, governments are spending billions of dollars to subsidize electric vehicles. These subsidies include state and federal tax credits for purchasing ZEVs and programs to subsidize the installation of vehicle-charging infrastructure in businesses, households, and along highways. Several states also have mandated the sale of ZEVs. For example, an executive order signed by California governor Jerry Brown in January requires 5 million ZEVs to be on the state’s roads and highways by 2030.
Will these subsidies and programs accomplish their objectives? And at what cost? A review of the literature finds few cost-benefit studies on these key questions.
Editor’s note: A corrected version of this report has been posted. Changes can be found here.
- Broad-based adoption of ZEVs will increase overall emissions of sulfur dioxide, oxides of nitrogen, and particulates, compared with the same number of new internal combustion engines. The simple fact is that, because of stringent emissions standards and low-sulfur gasoline, new gasoline-powered cars and trucks today emit very little pollution, and they will emit even less in the future.
- While new ZEVs will reduce CO2 emissions compared with new internal combustion vehicles, the overall reduction will be less than 1% of total forecast energy-related U.S. CO2 emissions through 2050. That reduction will have no measurable impact on world climate—and thus the economic value of CO2 emissions reductions associated with ZEVs is effectively zero.
- Subsidies for ZEVs and the required infrastructure to support them benefit the higher-income consumers who can afford to purchase them at the expense of lower-income consumers who cannot. In California alone, the total cost of ZEV subsidies, including federal tax credits and state rebates for ZEV purchases, as well as subsidies for private and public charging infrastructure, is likely to exceed $100 billion.
______________________
Jonathan A. Lesser, PhD, is the president of Continental Economics, an economic litigation and consulting firm. He is the author of the new report, Short Circuit: The High Cost of Electric Vehicle Subsidies.
Executive Summary
Many proclaim that the days of the internal combustion engine are numbered because of emerging “zero-emissions vehicle” (ZEV) technologies, especially battery-powered electric vehicles, which supposedly offer better performance, lower cost, and, most important, “emissions-free” driving. In order to accelerate the internal combustion engine’s forecast demise, billions of dollars are being spent to subsidize these electric vehicles and the charging infrastructure needed to support them.
These subsidies include state and federal tax credits for purchasing ZEVs; programs that subsidize the installation of vehicle-charging infrastructure in businesses, households, and along highways; and programs to subsidize the installation of residential and commercial solar photovoltaic systems for charging battery-powered vehicles. Additionally, state utility regulators have approved programs by local electric utilities to install charging-system infrastructure. Some states have encouraged ZEV purchases by granting these vehicles special access to carpool lanes, even when driven alone.
Several states have implemented ZEV mandates as well as subsidies. For example, California, where almost half of all ZEV vehicles in the U.S. have been sold, requires that 22% of all new vehicles sold in 2018 be ZEVs. A January 2018 executive order signed by California governor Jerry Brown requires 5 million ZEVs to be on the state’s roads and highways by 2030. That same executive order also mandates 200 hydrogen fueling stations and 250,000 ZEV charging stations by 2025.[1]
The primary stated rationale for ZEV subsidies and mandates, such as those set out in Governor Brown’s executive order, is to reduce air pollution and carbon dioxide (CO2) emissions. Other rationales include improved “energy security” through reduced imports of crude oil and speeding a transition to a low-carbon, “green” economy.[2]
Will these programs accomplish their objectives? And at what cost? A review of the literature finds few cost-benefit studies on these key questions. Moreover, policymakers have rarely examined whether these subsidies are equitable, given that it is mostly higher-income individuals who purchase ZEVs
Introduction
Recently, predictions of the impending demise of internal combustion vehicles have become commonplace. For example, in August 2017, The Economist proclaimed that “the internal combustion engine’s days are numbered,” owing to rapid advances in battery technology.[3] The Washington Post stated that “when future auto historians look back, they may pinpoint 2017 as the year electric vehicles went from a promising progressive fad to an industry-wide inevitability.”[4] Toyota’s director of research and development similarly stated that the internal combustion engine will be gone by 2050, replaced with battery-powered and hybrid vehicles.[5] But not everyone is convinced.[6] In 2019, Mazda will introduce the most thermally efficient gasoline engine in history, which the automaker claims will be cleaner than an electric car—because most electric cars are charged using electricity generated from fossil-fuel plants.[7]
Several countries plan to slay the internal combustion engine administratively by banning the production and sale of vehicles powered by those engines. Norway and the Netherlands have announced that they will ban the sale of internal combustion vehicles (ICVs) by 2025; Britain and France have announced that they will do so by 2040.[8] India intends to allow only sales of electric vehicles starting in 2030.[9] Even Germany, where the internal combustion engine was born, has contemplated a ban on ICV sales.[10]
While the U.S. has not proposed any nationwide ban, legislation introduced in California would ban the sale of all ICVs in the state by 2040.[211] Although that legislation remains in limbo, California governor Jerry Brown’s January 2018 executive order requires 5 million zero-emissions vehicles (ZEVs) to be on that state’s roads and highways by 2030.[12] It also mandates 200 hydrogen fueling stations and 250,000 ZEV chargers by 2025.[13] Governor Brown’s earlier 2012 executive order required 1.5 million ZEVs by 2025.[14]
In fact, California has been attempting to accelerate a transition to ZEVs for almost 30 years. In 1990, the state initiated its first ZEV mandate, which required a minimum percentage of new vehicle sales sold in the state to be ZEVs and established a program of tradable credits that can be used by automakers to meet the mandate.[15] (Nine other states have subsequently adopted similar mandates.) In 2018, for example, the mandate requires a minimum of 22% of all new vehicle sales in the state to be ZEVs.[16] (The system of tradable credits is similar to emissions credits under the Clean Air Act, whereby electric generators that reduce power plant emissions at a relatively low cost sell excess credits to other generators for which reducing those emissions is more costly.) The ZEV mandate and emissions credit schemes are a direct subsidy for ZEV manufacturers, which profit by selling ZEV credits to other automobile manufacturers. Tesla, for example, earned $831 million from the sale of ZEV credits during the three-year period 2015–17, including more than $360 million in 2017.[17]
ZEV purchasers also receive various subsidies. These include a federal tax credit up to $7,500; state rebates to consumers and businesses for both the vehicles and the charging infrastructure they require; construction of charging stations along roadways and at public locations such as parks and government offices; and even preferred access to high-occupancy vehicle (HOV) lanes.
The oft-stated rationale for ZEV subsidies and mandates is to reduce air pollution and greenhouse gas (GHG) emissions, primarily carbon dioxide (CO2). Other rationales include improved “energy security” associated with reductions in imports of crude oil and a transition to a lower-carbon “green” economy. For example, Governor Brown’s January 2018 executive order affirms that increasing the number of ZEVs “will strengthen the economy, improve air quality and public health, lower fuel costs for drivers and reduce the state’s dependence on fossil fuels.”[18]
Proponents of ZEV subsidies argue that the benefits of reduced air pollution and GHG emissions will far exceed the costs of the subsidies. They further justify the subsidies based on the accompanying positive “network” externalities. In other words, subsidizing ZEVs and related infrastructure reduces costs and accelerates ZEV adoption by consumers, thus increasing nonmarket benefits for everyone.[19] An analogy is the historical subsidies for rural telephone lines and, more recently, high-speed Internet service. By expanding the population that telephones could (and the Internet now can) access, the value of the entire network increases.
Subsidy skeptics are less sanguine about the value of the claimed pollution reductions and network benefits. Furthermore, they raise concerns about fairness and equity, because most ZEVs have been purchased by affluent consumers.[20] As Daniel Gross wrote in a 2015 article for Slate:
“Many green-related public benefits flow almost exclusively to individuals who are already well off and don’t need the help.... From an environmental, social, and economic perspective, it would be smarter to subsidize the purchase of super-efficient, modestly priced gas and diesel vehicles by middle- and lower-income people than to give hedge-fund managers $7,500 for buying a Tesla.[21]”
Indeed, a 2013 analysis found that Tesla buyers had an average household income of $293,200.[22] A 2015 study found that buyers of the far lower-cost Ford Focus electric vehicle nevertheless had an average household income of $199,000.[23] By contrast, 2015 median household income in the U.S. was $56,516.[24]
Tesla CEO Elon Musk has staked the future of his company on producing a lower-cost ZEV, the Tesla Model 3, which sells for about $35,000. But Tesla’s stated goal to build 500,000 Model 3s annually is far from being realized. Most recently, Moody’s lowered the company’s bond rating (it was already at “junk” status) to reflect the lag in production.[25] Tesla closed out the first quarter of 2018 short of its goal of producing 2,500 units a week, far below the 10,000 weekly number needed to achieve the 500,000 annual mark. Early in the second quarter, production was temporarily halted as the company made assembly-line adjustments, which it said would permit production of as many as 6,000 units a week by the end of the second quarter.[26]
Laws that mandate increasing percentages of ZEV sales or ban the sale of internal combustion vehicles outright will ensure a robust, if only artificial, market for ZEVs. Whether such laws make economic and environmental sense is another question. In fact, despite the popularity of ZEVs—and ZEV-related subsidies—among policymakers, there are no studies that have estimated the costs and benefits of those subsidies based on projections of future ZEV sales nationwide. This report is an attempt to do so.
The organization of this report is as follows:
- Section I examines the current state of the ZEV market.
- Section II provides a summary of the different subsidies for ZEVs, as well as estimates of their direct and indirect costs.
- Section III estimates the air pollution and GHG reductions of ZEV-promoting policies and the economic value of those reductions.
- Section IV addresses the implications of a complete transition to ZEVs on electricity demand and supply and the challenges of meeting that demand solely with “emissions-free” wind and solar power.
- Section V considers the equity impacts of ZEV subsidies and related infrastructure costs. Currently, ZEVs, as well as the infrastructure needed to support them, primarily benefit upper-income individuals. However, the costs of these subsidies are borne by all taxpayers, businesses, and, especially, lower-income individuals. This is particularly true for scenarios in which ZEVs are charged with consumer-generated (called “behind-the-meter”) solar power, from photovoltaic panels installed on homes and businesses, which are themselves subsidized through federal and state tax credits, as well as electric utility “net-metering programs.” These equity issues will likely assume greater importance as ZEV sales grow.
- Section VI offers conclusions.
I. The ZEV Market Today
Despite their rapid growth in sales, ZEVs remain a minute fraction of the U.S. automobile market. Over the seven-year period 2011–17, sales of all ZEVs totaled about 722,000, versus non-ZEV vehicle sales of more than 110 million (Figure 1), or less than seven-tenths of 1% of all sales.[27] In 2017, sales of all ZEVs totaled about 190,000, just over 1% of the 17.1 million passenger cars and light trucks sold that year.[28]
Hydrogen-powered fuel-cell vehicles (FCVs) remain a tiny niche among ZEVs, with sales totaling about 6,400 worldwide.29 Through December 2017, a total of 3,084 hydrogen FCVs were sold in the U.S., virtually all in California,[30] or about one-third of 1% of all ZEV sales. In 2017, U.S. FCV sales totaled 1,862. In January 2018, Toyota announced that it had reached 3,000 worldwide sales of its FCV, the Mirai.[31]
But absent significant improvements in hydrogen fuel-cell and hydrogen production technologies, it is unlikely that sales of FCVs will overtake sales of plug-in hybrid vehicles (PHEVs) and battery-powered electric vehicles (BEVs), owing to better and lower-cost batteries. Today, some electric vehicles, such as the Tesla Roadster, offer performance that rivals, if not exceeds, the highest-end sports cars.[32]
California, with just over 356,000 ZEVs sold from 2011 through 2017, accounted for roughly half of all ZEV sales in the country (Figure 2).
Despite their rapid growth in sales, ZEVs are projected to remain a small fraction of the overall vehicle stock. The U.S. EIA’s Annual Energy Outlook 2018, for example, predicts that ZEVs will constitute only about 18% of the total stock of cars and light trucks by 2050 (Figure 3).[33]
ZEV sales have been encouraged by numerous federal, state, and local subsidies. For example, since 2010, the U.S. government has provided a $7,500 federal tax credit for BEVs and a $2,500 credit for PHEVs.[34] Additionally, many states provide both cash rebates and tax incentives for ZEV purchases, as well as direct subsidies for installing residential and commercial battery-charging stations and public charging stations. Some states even provide special access to HOV lanes for ZEVs.[35] Various local communities also provide subsidies for charging infrastructure.
For example, California offers a rebate of $2,500 for BEVs, $1,500 for PHEVs, and $5,000 for FCVs.[36] The state has also spent hundreds of millions of dollars installing charging-system infrastructure along major highways, and it grants HOV-lane access to all ZEVs. What’s more, California’s electric utilities offer subsidies for installing home and business charging stations, as well as provide special electric rates for charging ZEVs. Meanwhile, various California cities, including Los Angeles, offer their own subsidies for ZEV charging infrastructure.[37]
There is little doubt that all these various subsidies have spurred ZEV sales.[38] Perhaps the clearest evidence of this is Georgia. In July 2015, Georgia eliminated its $5,000 tax credit for all ZEVs. Prior to that month, Georgia had accounted for 17% of all U.S. ZEV sales, more than any other state except California. After the tax credit was eliminated, sales of all ZEVs plummeted by almost 90%, especially among lower-priced ZEVs, such as the Nissan Leaf.[39]
Automobile manufacturers also have an incentive to sell ZEVs because they are exempt from the GHG emissions mandate established in 2010 for gasoline- and diesel-powered vehicles under the Corporate Average Fuel Economy (CAFE) standards.[40] In other words, for purposes of meeting CAFE standards, ZEVs are treated as “zero-pollution” vehicles, even if the electricity they use is produced from fossil fuels.
II. A Compendium of ZEV Subsidies
Currently, 38 states, the District of Columbia, and the U.S. government offer various programs that directly or indirectly subsidize ZEVs.[41] As noted, the U.S. government offers a tax credit up to $7,500 for ZEVs. Thirty-one states offer incentives for purchasing ZEVs or charging equipment, including seven states that offer rebates, tax credits, or both.[42] Other state subsidies include the development of charging stations along major highways that can be used free of charge.[43]
Private incentives, typically offered by electric utilities, are provided in 23 states. Most of these incentives offer discounts for the equipment needed to charge ZEVs at home or at work. The costs of those programs, which must be approved by state utility regulators, are paid by all electric ratepayers. (In addition, several electric utilities provide direct subsidies for the purchase of electric vehicles.) Moreover, many electric utilities provide additional subsidies for customer-owned, “behind-the-meter” solar photovoltaic (PV) systems, which can be used to charge BEVs and PHEVs. Several utilities, such as Pacific Gas & Electric Company (PG&E) in California, have also built charging stations throughout their service territories.[44] Again, the costs of these subsidized programs are paid for by all electric customers, based on traditional methods for allocating costs among residential, commercial, and industrial customers.[45]
In addition to these direct and indirect subsidies for ZEVs and related infrastructure, several states allow ZEVs preferential access to HOV lanes. As discussed below, the estimated economic value of such access is large and can be a motivating factor for individuals considering the purchase of a ZEV. Moreover, because owners of BEVs and FCVs do not use gasoline, they do not pay gasoline taxes to fund highway maintenance.[46] (Owners of hybrid PHEVs pay reduced amounts of taxes.)
Thus, an initial policy question is: What do these subsidies cost? The answer is: billions of dollars.
The Federal ZEV Tax Credit
The federal tax credit for ZEVs—BEVs and PHEVs— took effect on January 1, 2010. The tax credit starts at $2,500 for purchases of new vehicles with a minimum battery capacity of 5 kilowatt-hours (kWh) and increases by $417 for every additional kWh of battery capacity, up to a maximum of $7,500 (corresponding to a battery capacity of 17 kWh). For example, the 2018 Nissan Leaf has a capacity of 40 kWh, and thus receives the maximum $7,500 amount. The most popular BEVs manufactured today, although not all PHEVs, have capacities larger than the 17-kWh minimum required for the top tax credit (Figure 4).
The rightmost column in Figure 4 lists energy efficiency in terms of electricity use per mile, rather than miles per kWh.[47] The lower the electricity use per mile, the greater the energy efficiency of a BEV. As shown, the claimed energy-usage values range between 0.23 and 0.34 kWh/ mile and average 0.28. However, as discussed below, ZEV energy-usage values under actual driving conditions can be much higher, much as EPA mileage stickers on ICVs fail to reflect actual driving conditions.
The federal ZEV tax credit applies in full to the first 200,000 vehicles sold by a manufacturer that are registered in the U.S. (Sales in foreign countries do not count toward the 200,000 limit.) The tax credit is reduced by 50% at the beginning of the second full calendar quarter after that milestone is reached. At the beginning of the fourth full calendar quarter after that milestone is reached, the tax credit is reduced to 25% of its original level for an additional six months, after which the credit expires.
For example, suppose a manufacturer reaches the 200,000 milestone on February 15, 2018 (during the first quarter of 2018). The full tax credit continues until July 1, 2018 (the beginning of the third quarter of 2018), when it is reduced by 50% of the full credit. It remains at that level through the end of December 2018. On January 1, 2019, the credit is reduced to 25% of the original amount until June 30, 2019, and then expires. Thus, in this example, a tax credit continues to be available for 16.5 months after the manufacturer reaches the 200,000-vehicle milestone.
Tesla, which is estimated to have sold about 156,000 BEVs in the U.S. from 2011 through 2017,[48] is likely to be the first manufacturer to lose the federal tax credit, followed closely by General Motors, which sold about 162,000 ZEVs during that period.[49] (Although GM has sold slightly more ZEVs than Tesla, Tesla’s sales have been increasing at a faster pace, and, hence, the company is expected to lose its federal tax credit before GM.) Based on reported sales of all ZEVs during the period and the federal tax credit for each make/model, federal tax credits received by ZEV purchasers totaled about $4.7 billion through 2017 (Figure 5).
Of that $4.7 billion total, purchasers of BEVs received about $2.87 billion, while purchasers of PHEVs received about $1.80 billion.
Total tax credit payments during the phaseout period may exceed the credits paid for the first 200,000 vehicles, depending on how many ZEVs are sold by a manufacturer during the phaseout.
Let’s first determine what the total tax credits might be if each manufacturer sold 200,000 ZEVs. Using the current tax credit for the different models sold by each manufacturer and the sales of each model during 2011– 17, we can calculate the weighted average tax credit by automaker. Assuming the same mix of vehicles sold continues, the total tax credit for each manufacturer can be estimated as the weighted average tax credit multiplied by 200,000, the milestone sales limit for maximum credits (Figure 6). As shown, if each ZEV manufacturer sells 200,000 vehicles, the tax credit will total nearly $17.7 billion. In fact, this value may underestimate the total credits because the weighted average values include older models with smaller battery capacities than current ZEVs.
It is difficult to predict how many ZEVs each manufacturer will sell in the year after reaching its respective 200,000-vehicle milestone. Suppose, however, that each reaches the sales milestone in the middle of a quarter (February 15, May 15, etc.) and then sells 10,000 ZEVs per month thereafter. In that case, using the weighted average tax credit values shown in Figure 6, the additional tax credit payments would be about $8.0 billion after reaching the milestone, and federal tax credits for ZEVs would total $25.6 billion. Because no sales cap applies to tax credits after the milestone, total tax credits paid following the milestone may exceed the credits paid in reaching it.
Individual State ZEV Rebates
In addition to the federal tax credit, seven states offer rebates for the purchase of ZEVs, in amounts ranging from $2,000 to $5,000 (Figure 7).
For example, through January 31, 2018, California paid out just over $514 million in rebates associated with purchases of just over 232,000 ZEVs, or over $2,200 per ZEV, including roughly $355 million for BEVs and $140 million for PHEVs.[50] Given Governor Brown’s January 2018 executive order requiring 5 million ZEVs by 2030, the ultimate cost of these rebates could exceed $10 billion by 2030.[51] Although the rebates depend on state government funding, the rebate fund has been replenished repeatedly.
The expenditures for ZEV rebates in other states are likely to be far less. As shown in Figure 7, several states have placed expenditure caps on rebate programs, such as the $55 million cap for New York’s Drive Clean program.[52] (Whether that program will receive additional funding is unknown.) In the other states that offer rebates, relatively few ZEVs have been sold, and thus the rebates will be small relative to California. For example, even if we assume that annual ZEV sales in each of these states equal total sales from 2011 through 2017, non-California rebates will likely total less than $500 million through the end of 2020.
Subsidies for Charging Stations
Until the end of 2016, the U.S. government offered a 30% tax credit on electric-vehicle charging stations. There are no available data on how many individuals claimed the 30% credit for installing a residential charging station. However, we can estimate a range of values for this credit, based on the costs of residential stations.
According to HomeAdvisor, the median cost of a simple residential ZEV charging station was $647 in 2017.[53] The cost has declined over time—a 2015 study by the Idaho National Laboratory, for example, estimated an average cost of $1,357 for residential charging stations for 2011–13.[54] Although some BEV owners may rely solely on public or workplace charging stations, most will install a charging station.
From 2011 through 2016, about 517,000 BEVs and PHEVs were sold (excluding fleet and government sales). If every BEV and PHEV owner also installed a residential charging station, at an average cost of $1,000, the federal tax credits would have totaled about $155 million.[55] Presumably, the actual value is less than this. But even if not, the charging station subsidies are small, compared with federal tax credits for purchasing ZEVs themselves.
In addition to the now-expired federal tax credit for ZEV charging stations, many states offer subsidies for residential and commercial ZEV charging stations in the form of tax credits and rebates.[56] Louisiana, for example, offers a tax credit of 36% of the cost of residential charging stations, up to a maximum of $1,500. Missouri provides a 100% tax credit up to $1,500 for residential charging stations.
Commercial and public charging stations are far more costly, especially direct-current (DC) fast-charging stations, which can charge a vehicle’s batteries to about 80% of capacity in 30 minutes. (By contrast, using a 110-volt Level 1 residential charger, which can be plugged in to a wall outlet, a standard Tesla Model 3 with a 50 kWh battery pack would require more than 35 hours to charge fully.)[57]
Public charging stations primarily use 240-volt (Level 2) chargers. They can charge a Tesla Model 3 in 6.5 hours. The fastest charging systems available are called Direct Current Fast Chargers (DCFCs). Commercial DCFCs have a power output of 50 kW, which can provide about 90 miles of range for a typical ZEV in about 30 minutes.[58]
The Challenge of BEV Charging Times
An ICV’s gas tank can be refilled in two minutes or so, far faster than even a DCFC can recharge a ZEV. Although Tesla has a proprietary network of 120-kW fast chargers that can provide the 90-kWh Model S with enough electricity in 30 minutes to travel 170 miles—and a full recharge in 75 minutes—that’s still a lot of time, especially on a long-distance trip. This issue has created what some call “charging time trauma,”[59] which may impede future ZEV sales.
Even the newest generation of chargers, which operate at 320–350 kW, will still take 15–20 minutes to recharge a ZEV. Moreover, such large-capacity chargers will require new distribution system infrastructure to handle the loads. Another issue: almost no ZEVs on the road today can charge at such a high power level.
Although various new battery technologies promising lower costs, greater capacity, and far faster charging times have been announced repeatedly, their commercialization remains elusive.[60] Thus, it would appear that “charging time trauma” will continue to affect many ZEV owners for the foreseeable future.
Many electric utilities have received regulatory approvals to install public charging station networks, whose costs are allocated to all utility ratepayers based on standard regulatory cost-allocation techniques among residential, commercial, and industrial customers.[61] For example, a December 2016 decision by the California Public Utilities Commission allows PG&E to install 7,500 Level 2 charging ports and 100 DCFC charging stations in the first phase of developing a charging infrastructure throughout its service territory, at an overall estimated initial cost of $160 million.[62] Other electric utilities are pursuing similar programs. In New York, for example, Consolidated Edison is partnering with New York City to install charging stations in all five boroughs.[63]
Data published by Southern California Edison (SCE) for its $22 million “Charge Ready” program show that, through December 2017, the average installed cost of a Level 2 charging station is $214,550 for a typical 15-port public station, or about $14,000 per charging port.[64] The average cost of a DCFC is estimated to be more than $50,000 per port. For example, Austin Energy’s two-unit Seaholm public DCFC had a reported installed cost of $105,823, or $52,912 per port.[65]
Estimated Costs of a Nationwide Charging Infrastructure
Several studies have estimated the number of nonresidential charging stations that will be required to serve projected numbers of ZEVs. For example, a 2017 study prepared by the University of California–Berkeley’s Center for Law, Energy & the Environment (CLEE) estimated that between 125,000 and 220,000 public charging stations will be needed in California alone by 2020.[66] A March 2018 study prepared by the California Energy Commission (CEC) estimates that between 229,000 and 279,000 public charging stations will be needed in the state by 2025.[67] Nationwide, the number of charging stations needed will be far larger.
At the end of 2017, there were just 12,000 public charging stations in California, of which 1,500 were DCFCs.[68] The remainder were Level 2 charging stations. In the continental U.S., there were just over 47,000 public charging stations at the end of 2017, of which 6,288 were DCFCs.[69] By comparison, in 2012, there were 10,100 gasoline stations in California, down from 14,200 in 1996, and about 156,000 nationwide (Figure 8).[70]
Using the $14,000-per-port average cost and the 15-port average size of Level 2 public charging stations, the cost to install 125,000–220,000 such stations in California, as estimated by the CLEE report, is between $26 billion and $46 billion. Those costs would be borne primarily by California electric ratepayers. The cost to install the 229,000–279,000 charging stations that the CEC estimates will be required is between $48 billion and $59 billion.
Nationwide, a 2017 study prepared by the National Renewable Energy Laboratory (NREL) for the DOE Office of Energy Efficiency and Renewable Energy estimated that the charging needs for an assumed 15 million BEVs in 2030 would require 8,500 DCFCs across the country and about 600,000 Level 2 public charging stations. Using the data from the SCE Charge Ready program, 600,000 Level 2 chargers would cost $84 billion. The cost of installing 8,500 DCFCs, even if they have just two ports, such as the Austin Energy Seaholm project, would be about $900 million.
These U.S. figures, however large, likely underestimate the total number of charging stations needed. The NREL study assumes that 83% of BEVs will be located in cities with populations of at least 50,000 and that 88% of all BEV owners will charge their vehicles at their homes.[71] However, in many large cities, the percentage of single-family homes relative to the total housing stock is much lower. In 2015, about 36% of the housing units in San Francisco were single-family homes, compared with 63% nationwide.[72] In Los Angeles, 36% of the housing units were single-family homes that year;[73] in New York City, less than 32%.[74] To the extent that fewer city dwellers live in single-family homes, cities will likely need to provide extra public charging stations beyond the NREL estimate to accommodate ZEV owners who cannot install residential systems, raising overall costs.
In addition to receiving funding from electric utility ratepayers, many public charging stations are directly subsidized by taxpayers. For example, the Los Angeles Department of Water and Power’s “Charge Up L.A.!” program is designed to encourage installation of charging stations at businesses and apartment buildings. The $21.5 million program offers a subsidy of $4,000 per installed charger. The program is set to expire on June 30, 2018.[75] Sonoma County, in northern California, offers grants for development of public charging stations along highways, in parks, and at county buildings.[76] Similarly, Nevada has a program to subsidize charging stations along its highways. That program, called the Nevada Electric Highway, aims to develop a statewide network of charging systems by 2020.[77]
HOV Access
Six states—California, Florida, Hawaii, Maryland, Nevada, and North Carolina—offer ZEVs special access to HOV lanes.[78] This access allows single-occupancy ZEVs to use the HOV lanes, in contrast to requirements that other users have two (and, in some cases, three) or more occupants. In all but Nevada, this access is only temporary. In California, the preferential access expires January 1, 2019, for vehicles sold before January 1, 2017. Owners of vehicles sold in 2017 and 2018 can reapply and extend their access until January 1, 2022. For ZEVs sold in 2019 or later, access is granted until January 1 of the fourth year after the purchase date.[79] Special access expires in Florida, Maryland, and North Carolina on June 30, 2019, and on June 30, 2020, in Hawaii.[80]
Preferential HOV access is another incentive to encourage purchases of ZEVs. Although such access does not have a direct monetary cost, it has an indirect cost on other HOV users, whose travel times will increase. Moreover, preferential access can be thought of as a subsidy based on the avoided penalties for illegally using an HOV lane. Previous research has shown that, because commuters value their time highly, preferential access is potentially worth thousands of dollars per ZEV owner, based on the savings in commuting time.[81] For example, a 2014 study of California’s Clean Air Access program showed that drivers would have been willing to pay $5,800 for six years’ access to HOV lanes.[82]
Special Fees for ZEVs
Recognizing that ZEVs do not pay fuel taxes that support road maintenance (or, in the case of PHEVs, pay reduced amounts of tax), 17 states now impose additional licensing or registration fees on these vehicles.[83] For example, as of January 1, 2018, Minnesota charges ZEVs an additional $75 annual vehicle registration fee.[84] As of January 1, 2020, California will impose an additional $100 annual registration fee on ZEVs with a model year 2020 and later.[85] Thus, if 500,000 model year 2020 ZEVs are sold in California, their owners will pay a total of $50 million in extra registration fees. However, there is still no recouping of lost federal gasoline tax revenues avoided by ZEVs.
The difference between these registration fees and forgone fuel taxes will depend on state fuel tax rates and the distance that a typical ZEV owner drives each year. For example, in 2017, California’s state gasoline tax was increased to 41.7 cents per gallon. If a typical ZEV owner drives 10,000 miles per year and would otherwise have purchased a vehicle with an average fuel efficiency of 30 miles per gallon, the state would forgo $139 in gasoline taxes. For the ZEV fee to fully compensate for the forgone taxes, the gasoline vehicle would need to have an average overall fuel efficiency of 41.7 miles per gallon, a higher efficiency than almost all ICVs today.
III. Do Policies That Promote ZEVs Produce Environmental Benefits?
The oft-stated primary objective of programs to promote ZEVs is to improve environmental quality by reducing air pollution and GHG emissions. However, the actual benefits depend on a number of factors, including: (i) the fuel efficiency of internal combustion engines and their emissions control technologies; and (ii) the emissions from the power plants that generate electricity for BEV and PHEV batteries.
Crucially, there is another aspect in assessing the emissions-reduction impacts of subsidies: the degree to which they encourage long-term replacement of internal combustion vehicles with ZEVs. For example, if, as The Economist argues,[86] replacement of internal combustion vehicles with ZEVs is inevitable, the actual environmental benefits will be less than if ZEVs would not replace internal combustion engines but for the programs themselves. In other words, if one believes that ZEVs will inevitably replace internal combustion vehicles by, say, 2050 (i.e., 100% saturation), but the various subsidies accelerate that transition to, say, 2040, the subsidies simply accelerate by 10 years the air pollution reductions that would take place regardless. In that case, the benefits would be limited to the incremental air pollution reductions achieved during that 10-year period (Figure 9, Area A).
On the other hand, if one believes that ZEVs would never fully replace internal combustion vehicles without the existence of the various subsidies and instead would never rise above some market share (ZEVmax), the benefits from the avoided pollution would accrue over the long term. In this case, there would be additional environmental benefits over time (Figure 9, Area B).
In both cases, the benefits are offset by the additional costs of an uneconomic technology plus the costs of the subsidies themselves. Put another way, to justify ZEV subsidies from an economic standpoint, the value of the avoided pollution and GHG emissions would have to be greater than the additional costs of the subsidies.
Thus, if one believes that subsidies merely hasten an inevitable transition from fossil-fuel vehicles, society is simply accelerating benefits that would be realized anyway, and the net benefits (Area A) will be relatively small compared with the case that the transition would never take place but for the subsidies themselves (Area B). Although the potential environmental benefits in the latter case would be larger than the former, so would the costs, because the latter case means that ZEVs would never be economically competitive with ICVs.[87] From a policy perspective, the distinction matters because of the differing impact on projected future benefits.
Estimating Changes in Air Pollution Emissions[88]
As discussed in Section I, EIA Annual Energy Outlook 2018 forecasts that ZEVs will account for about 18% of the total U.S. vehicle stock by 2050. Of course, this prediction could change, owing to many factors, including improvements in battery and ICV technology, as well as changes in their costs; the costs of battery inputs, especially cobalt, which is used to manufacture lithium-ion batteries; the retail cost of electricity; and changes in the price of motor fuel, which will be affected by changes in crude oil prices, changes in fuel demand, and fuel taxes levied by the states and the federal government.
Forecasting the quantity and value of avoided emissions due to accelerated adoption of ZEVs is analytically complex. A number of studies have attempted to do this for individual vehicles, including studies that have estimated emissions over a vehicle’s entire life cycle.[89]
Such calculations require estimating emissions rates for vehicles, the average number of miles they are driven (“vehicle miles traveled,” or VMT), and the nonmonetary (external) costs of various pollutants. All these values vary by geography. For example, average VMT in rural areas typically exceeds VMT in urban areas. However, the external costs are typically greater in urban areas because of higher population density. For example, the Victoria Transport Policy Institute estimated average air pollution impacts to be 4.0 cents/ mile for all ICVs and 1.0 cent/mile for all ZEVs (all estimates in 2007$), based on specific estimates of pollution costs in Europe.[90] External costs in the U.S. would likely be lower because the U.S. has a lower population density. A 2008 study estimated that health-related costs of U.S. ICVs were between 0.2 cent/mile and 2.5 cents/mile, depending on the vehicle.[91]
Moreover, these values do not reflect current U.S. tailpipe emissions standards, called the “Tier 3” standards, whose phase-in began in 2017 and will be completed by 2025.[92] The Tier 3 standards establish maximum tailpipe emissions for oxides of nitrogen (NOx) and particulate emissions. In addition, the Tier 3 standards reduce the previously allowed sulfur content of motor gasoline from 30 parts per million (ppm) under the Tier 2 standards to 10 ppm.[93]
A detailed forecast of future vehicle emissions and nonmonetary costs by location is far beyond the scope of this report. Such an analysis would have to develop long-term forecasts of sales of ZEVs and ICVs by location, determine where such vehicles are driven (e.g., in cities, rural areas), the regional mix of electric generation over time, the patterns of air pollution emissions, and location-specific environmental damages.[94]
Instead, this report examines differences in nationwide air pollution emissions between the forecast number of ZEVs and the same number of ICVs, based on data in EIA’s Annual Energy Outlook 2018. Specifically, this report forecasts total ZEV emissions using: (i) EIA’s forecast of the number of ZEVs making up the light vehicle population (as shown previously in Figure 3); (ii) the agency’s forecast of VMT; (iii) a forecast of the energy efficiency of ZEVs; (iv) the agency’s forecast of the nation’s electric-generating mix; and (v) emissions standards for fossil-fuel-generating plants under the Clean Air Act for three key air pollutants—sulfur dioxide (SO2), NOx, and particulates—as well as CO2.
The analysis next derives comparable emissions forecasts for the same number of ICVs using EIA’s forecast of ICV mile-per-gallon efficiency and the Tier 3 emissions-per-mile standards. As part of the overall ICV emissions estimates, the analysis also includes emissions from petroleum refineries associated with manufacturing gasoline. The final step is to compare the external costs of these pollutants from ZEV and ICV emissions, based on published estimates of those costs.
Estimating Generating Plant Emissions Associated with ZEVs
The analysis estimates emissions associated with the electricity consumed by ZEVs using EIA’s forecast of the overall U.S. generating mix (coal, natural gas, nuclear, and renewables) by year, from 2016 through 2050. Using the forecast emissions from this generating mix, the analysis then calculates average emissions per kWh of electricity.[95]
Total electricity consumption of ZEVs equals the forecast number of ZEVs, multiplied by the average VMT per vehicle, multiplied by average per-mile electricity consumption.[96] The analysis calculates average VMT based on EIA’s forecast of total VMT for light-duty vehicles divided by the total light-duty vehicle stock.[97] The analysis assumes that average VMT is the same for a ZEV as for an ICV.
The reported energy consumption values for ZEVs, like EPA’s city and highway mileage estimates for new ICVs, do not consider the multitude of factors that can affect electricity consumption. Thus, the published energy consumption values in Figure 4 may underestimate electricity use by ZEVs. For example, extreme weather conditions, such as in upper Midwest and New England winters and desert Southwest summers, reduce electric vehicle range, both because of reduced battery efficiency and the need to divert battery power to heating and air conditioning.[98]
BEVs operate most efficiently only within a narrow temperature band. As temperatures diverge from that band—colder or hotter—BEV operating efficiency and mileage per charge decrease. Case in point: a 2014 analysis of driving ranges for the Nissan Leaf, with a battery capacity of 24 kWh, found that the Leaf achieved a maximum range of about 80 miles (0.30 kWh/mile) only within a temperature range between 60 degrees Fahrenheit (°F) and 70°F (Figure 10).[99] At an outside temperature of 0°F, common during winters in the upper Midwest, the Leaf’s range decreased to about 52 miles, equivalent to energy consumption of 0.46 kWh per mile, about 35% lower than the 80-mile maximum range. The vehicle has a similar range when the outside temperature is 100°F, common in summers in the Southwest.
Given the narrow band of temperatures associated with optimal consumption and range, the analysis assumes that the average BEV used 0.30 kWh/mile in 2016. As shown previously in Figure 4, the average energy efficiency of model year 2018 BEVs ranges from 0.23 to 0.34 kWh per mile, with an average value of 0.28. The analysis uses this average efficiency value for 2018. To incorporate the potential for improved battery and electric motor technology, the analysis assumes that energy consumption decreases at a constant rate to 0.24 kWh per mile by 2025 and further decreases to 0.20 in 2050. However, to be conservative, the analysis does not assume that battery performance degrades with age.
To estimate total electricity consumption by ZEVs, we must account for the fact that, in any given year, multiple vintages of ZEVs will be driven. For example, in 2020, model year 2016–19 ZEVs will still be on the road, along with 2020 models. To address this, the analysis calculates total electricity usage in any given year based on the remaining number of vehicles of each vintage. For example, in 2020, less than 100% of model year 2016 ZEVs will still be on the road, as will less than 100% of 2017 vehicles, and so forth, along with 100% of the 2020 vehicles. However, there is insufficient history with ZEVs to model a typical S-curve for ZEV retirements.[100] A 2017 study prepared for the Electric Vehicle Transportation Center on EV life-cycle costs assumed lifetimes of 5, 10, and 15 years. This analysis assumes a 10-year average life for a new ZEV.[101] With an assumed 10-year average life, by 2028, half of 2018 vintage vehicles will have been scrapped.
We can demonstrate the calculation using 2030 as an example. In that year, EIA forecasts a total of 8.4 million ZEVs in the U.S. and an average VMT for all light-duty vehicles of 11,512 miles/vehicle. Thus, total electricity use for ZEVs is based on the number of ZEVs of each vintage and each vintage’s assumed energy consumption value.[102] The resulting energy usage is 23.6 billion kWh, or 23.6 terawatt-hours (TWh). Incorporating EIA’s average value for transmission and distribution system losses of 5% increases the amount of electricity needed to 24.7 TWh.[103]
The analysis next calculates emissions from ZEVs, based on the forecast mix of electric-generating units and generation-related emissions. In 2017, coal-fired power plants accounted for about one-third of all electricity generation in the United States. EIA projects that, by 2050, coal’s share of total electricity generation will decrease to 22%. Similarly, EIA projects that nuclear power will decrease from about 20% of electricity generation today to about 12% by 2050.
The projected reductions in coal-fired and nuclear electricity generation will be replaced with natural gas generation and renewable energy. EIA forecasts that total natural gas-fired generation will increase by more than 50% from 2017 to 2050, and make up over 35% of total generation by then. EIA also projects that generation from all renewable energy resources (conventional hydroelectric power, solar, wind, biomass, etc.) will increase by about 150% over this period, accounting for more than 30% of all generation by 2050.[104] Thus, the EIA forecast assumes that the U.S. generation mix will become significantly “cleaner” over time and that average emissions per kWh will decrease.
Under the U.S. Clean Air Act, SO2, NOx, and particulate emissions are considered “criteria air pollutants,” which are associated with various health and environmental damages.[105] To calculate average emissions per kWh, we divide total emissions by total generation. In 2030, for example, EIA estimates total electric generation of 4,198 TWh[106] and total SO2 and NOx emissions of 1.25 million tons and 1.01 million tons, respectively.[107] Thus, the corresponding SO2 and NOx emissions rates are about 0.027 grams (gm) per kWh and 0.022 gm per kWh, respectively.[108] Multiplying the 24.7 TWh of ZEV-related electricity generation by these emissions rates implies ZEV-related emissions of about 7,300 tons of SO2 and 5,900 tons of NOx.
EPA distinguishes between two types of particulate emissions—PM2.5 and PM10—because they have different environmental impacts. (The numbers refer to the average diameter of the particles, in microns.) Of the two types, PM2.5 emissions pose the greater health concern.[109] However, because EIA Annual Energy Outlook does not forecast particulate emissions from generating plants, the analysis uses the 2014 EPA Emissions Inventory, the most recent data available, to determine average emissions from coal-fired and natural gas-fired generating plants.[110] Specifically, the 2014 EPA Emissions Inventory provides detailed PM2.5 and PM10 emissions data from electric-generating plants (Figure 11).
Using the data in Figure 11, average PM10 emissions from coal-fired power plants were 246.8 lbs/gigawatt-hour (GWh),[111] while average PM2.5 emissions were 185.4 lbs/GWh (PM10 emissions include all particles less than 10 microns in diameter, and thus include PM 2.5 emissions). Similarly, average PM10 emissions from natural gas-fired power plants were 44.2 lbs/GWh and average PM2.5 emissions were 42.7 lbs/GWh. Thus, the average emissions rate for natural gas-fired power plants is about one-sixth as large as for coal-fired power plants.
Under the National Ambient Air Quality Standards (NAAQS) of the Clean Air Act, new coal-fired power plants must limit overall particulate emissions to 0.09 lbs/MWh (90 lbs/GWh). New natural gas-fired plants have virtually zero particulate emissions.[112]
Therefore, total particulate emissions associated with ZEVs equals total ZEV electricity use times the average emissions rates. In 2030, for example, total particulate emissions would be just over 1,100 tons at the average 2014 emissions rates and about 350 tons if all generating plants meet the new NAAQS.
Estimating NOx, SO2, and Particulate Emissions from ICVs[113]
Although some early ZEV adopters may have retained their ICVs, as ZEV sales become more common, consumers presumably will decide to replace an existing vehicle with a new ZEV or a new ICV. Thus, to compare the pollution from the electricity generated to power ZEVs with pollution from ICVs, the analysis assumes that every ZEV purchase replaces an ICV.
To estimate emissions from new ICVs, the analysis assumes that these vehicles will be driven the same number of miles per year as ZEVs. Total emissions are then based on the corresponding emissions standards for new ICVs. As mentioned previously, the analysis also includes emissions from oil refineries in proportion to the share of gasoline that refineries produce relative to the total production of all petroleum products. That step is necessary in order to compare total emissions from the combustion of fossil fuels, either to generate electricity or to power ICVs. Although not a complete study of “life-cycle” emissions—which would also include emissions related to vehicle and battery manufacture, battery disposal, and so forth—the analysis provides a more accurate and conservative estimate of emissions from ICVs by including refinery data.
Tier 3 standards restrict average ICV NOx emissions to no more than 0.037 gm per mile in 2017 and 0.030 gm per mile by 2025. The Tier 2 and Tier 3 standards for sulfur content are expressed in ppm. These can be converted into equivalent SO2 values per gallon based on a standard equation.[114] The resulting gm per gallon values are 0.001605 for Tier 2 and 0.000535 for Tier 3. Dividing these by the average mile-per-gallon values for new vehicles of each vintage yields the annual SO2 gm-per-mile values.[115]
To account for tightening emissions standards from 2016 to 2025, the analysis calculates the average emissions rate per year using the same vintage-based analysis described previously for ZEVs, using an estimated 15-year average lifetime for ICVs.[116] Thus, by 2033, half of 2018 vintage vehicles are assumed to have been scrapped.
The analysis shows that, based on the forecast U.S. generating mix, NOx pollution from the generating plants supplying ZEVs will exceed NOx emissions that would be emitted by the same number of ICVs (Figure 12).
By 2050, annual NOx emissions from the electricity generated for ZEV use increases to about 15,500 tons, whereas NOx emissions from the same number of ICVs, including oil-refinery NOx emissions, would be just over 13,600 tons. Annual ZEV-related SO2 emissions increase to about 20,000 tons by 2050, whereas annual SO2 emissions from the same number of ICVs would be about 2,600 tons, less than 15% of the ZEV-related total. Thus, increased reliance on ZEVs increases NOx and, especially, SO2 emissions relative to the same number of ICVs. The cumulative additional emissions from ZEVs over the 2016–50 period are about 51,000 tons of NOx and 299,000 tons of SO2.[117]
To compare particulate emissions of ZEVs relative to the same number of ICVs, the analysis uses both the 2014 PM average emissions rates from existing generating plants and the new emissions standards (Figure 13). As shown in the figure, if we assume that all existing coal- and natural gas-fired generating plants meet the new NAAQS for emissions, then total forecast particulate emissions associated with the electricity needed for ZEVs eventually decline to about half those of ICVs. In 2050, for example, generation-related emissions are about 930 tons, while ICV emissions, including refinery emissions, are about 1,670 tons. Based on the NAAQS, from 2016 to 2050, ZEVs provide a cumulative reduction in particulate emissions of about 11,000 tons versus an equivalent number of ICVs.[118]
In contrast, if the 2014 average emissions rates remain constant for the entire forecast period, PM emissions from ZEVs will be greater than ICV emissions—almost 3,200 tons per year by 2050, with a cumulative difference of about 25,000 tons. The reality is likely somewhere in the middle, depending on the mix of plants that meet the NAAQS. Given the relative differences in the two emissions forecasts for generating plants shown in Figure 13, PM emissions associated with ZEVs likely will somewhat exceed those from the same number of ICVs.
CO2 Emissions Estimates
The analysis uses a similar approach to estimate CO2 emissions from ZEVs and ICVs. First, using the same EIA forecast, we divide total forecast CO2 emissions by total forecast generation to calculate average CO2 emissions/kWh. We then multiply this value by the forecast of ZEV electricity consumption. CO2 emissions of ICVs are calculated based on an emissions rate of 19.6 lbs CO2/gallon of blended gasoline,[119] the same average lifetime and vintage assumptions described previously, and EIA’s average miles-per-gallon estimates in each year, which are adjusted downward for actual on-road performance.
As shown, CO2 emissions from ZEVs will be lower than those of the same number of ICVs (Figure 14). Between 2018 and 2050, the net reduction in CO2 emissions provided by ZEVs is 896 million tons if EPA’s existing CO2 per-mile standards remain in effect. If the standard is revised, and assuming the 2021 standard is maintained until 2050, the net reduction in CO2 emissions would be about 1.175 billion tons. This reduction is small, about six-tenths of 1%, compared with EIA’s total forecast energy-related CO2 emissions over this period, about 196 billion tons.[125]
In summary, subsidies and mandates designed to accelerate migration from ICVs to ZEVs would result in greater emissions of criteria air pollutants—SO2, NOx, and particulates—but lower emissions of CO2. Thus, one of the key claims used to justify ZEV subsidies and mandates to replace ICVs—that they will reduce levels of criteria air pollutants—is unsupported. Although the analysis shows that ZEVs will reduce CO2 emissions relative to an equivalent number of ICVs, the reductions will have no impact on climate and, hence, no economic benefit. This will be true even if, as discussed below, ZEVs were powered using electricity generated only from renewable sources.
Estimating the External Costs of Additional NOx, SO2, and Particulate Emissions
Next, we turn to comparing the external environmental costs of the criteria air pollutant emissions of ZEVs relative to an equivalent number of ICVs for 2016–50. NOx, SO2, and, especially, particulate emissions are associated with human health problems. Particulate emissions have been implicated in heart and lung disease and what are called “premature” deaths. (The definition of a “premature” death can be nebulous.) Moreover, both NOx and SO2 react chemically with other compounds in the atmosphere to form particulates. In addition to adverse health impacts, NOX and SO2 are associated with adverse environmental impacts because they can increase acidity levels in water, leading to recreational and commercial fishing losses and losses to forests. Moreover, both pollutants can reduce visibility, thus damaging natural scenery. In a 2010 report, the National Research Council published estimates of the per-ton costs of these emissions (Figure 15).[127]
To calculate the overall monetary damages of the projected emissions of these pollutants, the analysis uses the mean values in Figure 15, as adjusted for inflation using the U.S. Federal Reserve Gross Domestic Product Implicit Price deflator (GDPIPD).[128]
As Figure 15 shows, the estimated per-ton damages for PM2.5 are much larger than the damages for PM10 emissions. As discussed previously, because EIA Annual Energy Outlook 2018 does not forecast particulate emissions, we use the percentage of PM2.5 emissions provided in the 2014 EPA Emissions Inventory to estimate an average PM emissions damage cost.
Specifically, as Figure 11 showed, for a coal-fired plant, 75% of the particulate emissions are PM2.5. For natural gas plants, the corresponding percentage of PM2.5 emissions are 97%. The annual damages from PM emissions are calculated based on total PM emsissions from coal- and natural-gas fired generators, and the percentage of PM2.5 emissions relative to total PM emissions in each year.
Assuming that the same ratios of PM10 and PM2.5 emissions hold for generating plants meeting the NAAQS, which restrict coal-fired plants to 90 lbs/GWh and natural gas-fired ones to virtually zero, we can derive estimates of the annual particulate emissions by using EIA projections of the mix of coal- and natural gas-fired generation. The resulting annual environmental damages for ZEVs increase steadily, to just over $168 million (2017$) by 2050, of which $136 million, about 81%, is associated with SO2 emissions; $29 million, about 17%, is associated with NOx emissions; and $3 million, about 2%, is associated with PM10 and PM2.5 emissions (Figure 16).[129]
By contrast, total damages associated with SO2, NOx, and particulate emissions from the same number of ICVs, including emissions from refineries associated with the production of gasoline, are predicted to increase to just $48 million per year (2017$) in 2050, less than one-third of the damages incurred to power ZEVs.[130]
On a present-value basis, over 2016–50, total damages from SO2, NOx, and particulates associated with ZEVs are $2.81 billion (2017$), while damages from the same number of ICVs (including refinery-related emissions) would total $730 million (2017$).[131]
IV. ZEVs and a Future with All-Renewable Generation
The findings that ZEVs will increase air pollution relative to an equivalent number of ICVs relied on EIA Annual Energy Outlook 2018 projections. As discussed previously, EIA forecasts that, by 2050, renewable resources, including hydroelectric, will account for about 30% of all generation, while natural gas will account for 35%.[132]
Some ZEV advocates point to renewable energy as the means to true zero-emissions vehicles.[133] Thus, one objection to the analysis in this report might be to claim that most ZEVs will be purchased in states with higher renewable portfolio standard (RPS) mandates, and thus less fossil-fuel generation. For example, California and New York both have stringent RPS mandates requiring 50% of all electricity generation from renewable resources by 2030,[134] as well as other mandates to reduce GHG emissions. Other states have similar CO2 reduction goals, and many have their own RPS mandates, which could reduce emissions per kWh.
Thus, states with the most stringent RPS mandates, such as California, might claim that ZEVs operating on their roads will be truly zero-emissions vehicles. As such, these states could, in theory, demonstrate that benefits of the resulting pollution reductions will exceed the cost of the subsidies and mandates to promote ZEVs.
If all electricity were produced from emissions-free generating resources (including nuclear power), air pollution associated with ZEVs would be zero (ignoring other life-cycle emissions, such as manufacturing). However, a future where ZEVs nationwide are charged with electricity generated solely from renewable resources faces significant economic and engineering obstacles.
A direct economic obstacle to broad-based adoption of ZEVs is that states with the most stringent RPS mandates also have the highest average retail electricity prices. Higher electric prices reduce the “fuel” savings of ZEVs relative to ICVs. For example, in 2017, the average price of electricity for residential customers in California was 18.24 cents/kWh and the comparable rate in New York was almost as high, at 18.04 cents/kWh, almost 50% higher than the average rate for the other contiguous U.S. states, 12.25 cents/kWh.[135]
Other states with stringent RPS mandates include Connecticut, which requires 23% of electricity from renewable resources by 2020; Massachusetts, which requires 25% by 2030, along with a requirement for 1,600 MW of in-state solar photovoltaics by 2020;[136] Rhode Island, which requires 38.5% by 2035; and Vermont, which requires 75% by 2032.[137] These New England states also have the highest average residential electric rates. In 2017, the average residential rate in New England as a whole was 18.93 cents/kWh, with Connecticut, at 20.31 cents/kWh, having the highest retail rate in the entire contiguous U.S.[138]
Absent additional state subsidies or increased taxes on gasoline, higher electric prices relative to gasoline will tend to reduce the “mainstream” demand for ZEVs. In other words, in contrast to ZEV enthusiasts, who can be characterized as “early adopters,” typical consumers will not purchase ZEVs unless they provide superior economic value to comparable ICVs.[139] Moreover, because the demand for gasoline will decrease as more individuals switch to ZEVs, the market price of gasoline also will tend to decrease, further reducing the economic benefit of purchasing a ZEV based on fuel savings.
One engineering obstacle to widespread ZEV adoption may be the weather because, as discussed previously, extreme weather conditions reduce the range of battery-powered vehicles, both because of reduced battery efficiency and the need to divert battery power to heat and air conditioning. Thus, sales of ZEVs in New England states, New York, and New Jersey may be less than expected as mainstream consumers find that the vehicles’ operating performance is less than anticipated while operating costs are greater than anticipated. Coupled with already-high electricity prices, the economic incentive to purchase ZEVs will be diminished in those states.
ZEV proponents also may claim that EIA renewable-generation projections, about 30% of all generation by 2050, are too pessimistic because the U.S. will be able to obtain all or most of its electric-generation requirements from nonpolluting renewable resources as wind and solar photovoltaic generation increases. Indeed, some studies, such as a widely cited 2015 article coauthored by Mark Jacobson of Stanford University, have claimed that, by 2055, the entire U.S. will be able to meet 100% of its electric needs solely with wind, solar, and hydroelectric generation at a relatively low cost.[140] But Jacobson’s analysis and findings were later found to be the product of invalid models and numerous modeling errors, as well as invalid and unsupported assumptions.[141]
Moreover, because of the inherent intermittency of wind and solar generation, they require extensive backup generating resources or energy storage. Consequently, when the cost of backup generation and storage is included, the costs of wind and solar generation are greater than those of fossil-fuel generation, especially natural gas-fired generation. For example, a recent study found that, while technically feasible, meeting 80% of electricity demand with renewables not only would require hundreds of billions of dollars in new transmission system investment but would also require hundreds of billions of dollars in energy storage investments alone.[142] That same study estimated that meeting 100% of electricity demand with renewable energy would require an investment of $1 trillion for storage.
Thus, mandates for complete reliance on renewables will require huge investments in backup resources, whether energy storage or gas-fired generation, and higher electricity costs. What’s more, coupled with mandates for ZEVs to represent larger shares of the entire light-duty vehicle stock and the resulting increase in electricity demand, RPS mandates will require still greater investment in higher-cost renewable generation. Those higher electric costs will further reduce the cost savings of ZEVs relative to ICVs.
Even building just enough new wind or photovoltaic capacity to meet ZEV needs will be very costly. For example, using the prior energy consumption assumptions about ZEVs and EIA’s VMT forecast through 2050, if ZEVs constituted the entire vehicle stock, ZEV-related electricity use would increase almost 10-fold in 2050, from about 74 TWh to 709 TWh, not including energy losses associated with charging stations.[143] The difference in electricity consumption, 635 TWh, represents a 13% increase in EIA’s total forecast for U.S. electricity consumption of 4,708 TWh and an 80% increase in EIA’s overall forecast growth in electricity consumption from 2016 through 2050.[144]
According to EIA data, the average capacity of onshore wind generation nationwide is about 30%.[145] At an average capacity factor of 30%, that is, producing electricity 30% of all hours of the year, generating 635 TWh of additional electricity from wind would require about 242,000 MW of additional wind capacity,[146] requiring a land area of more than 31,000 square miles,[147] roughly the area of South Carolina. At the end of 3Q 2017, total U.S. wind-generating capacity was about 90,000 MW.[148] Much of the capacity was built to take advantage of the federal wind production tax credit, which now stands at $23 per MWh for wind facilities that began construction before 2018.[149] The credit will be reduced for facilities built in 2018 and 2019, after which time it expires. The end of the tax credit for new wind facilities will reduce the profitability of new wind generation significantly.
Moreover, as my Manhattan Institute colleague Robert Bryce has documented, opposition to siting new land-based wind-generating facilities is increasing. Vermont, for example, has a virtual moratorium on any new wind developments. Opposition in New York State to new wind facilities has also grown, as it has in Maryland, Michigan, and even California.[150]
Solar PV requires less land than for wind but requires extensive backup storage to address the lack of generation at night and on cloudy days. For example, EIA estimated the average nationwide capacity factor for solar PV to be 27% in 2017, ranging from a low of 16.8% in January to a high of 35.7% in June.[151] These averages mask regional differences: lower capacity factors in New England and higher ones in the desert Southwest.
The need for battery storage becomes more important if most ZEV owners recharge their vehicles at home and at night. For example, if ZEV energy used 635 TWh per year, or about 1.73 TWh on an average day, then providing just half of that electricity from residential battery storage would require 0.86 TWh of battery storage. The installed cost for a Tesla Powerwall 2 residential storage unit, which can provide 14 kWh of electricity, ranges from $7,000 to $8,200.[153] Thus, supplying 0.86 TWh of electricity with Powerwalls would require more than 61 million units, at a cost between $427 billion and $500 billion.
Furthermore, as shown previously in Figure 4, the most popular BEV cars—the Tesla S 75 and S 100 and the Chevy Bolt—have battery capacities of 60 kWh to 100 kWh, meaning that a homeowner would need to install up to a half-dozen Powerwalls to store sufficient solar-generated electricity for nighttime recharging, at a cost that could exceed that of the ZEV itself. It seems unlikely that BEV owners would be willing to spend as much as or more than their vehicle’s cost on storage, plus pay for a behind-the-meter solar PV system.
Without battery storage, ZEV owners who also install residential solar PV must rely on the utility grid for backup. As I discuss in the next section, this reliance raises significant equity issues because lower-income consumers are likely to foot a disproportionate share of these additional costs.
If electric utilities added storage to address intermittent wind and solar generation, the calculations would be similar. Again, the costs would be borne by all ratepayers, further exacerbating the impacts on lower-income ratepayers, as is discussed in the next section. In summary, the current high cost of battery storage makes a 100% renewable-generation portfolio for ZEVs unrealistic.
A likely lower-cost way to provide true zero-emissions electricity for ZEVs would be to build new nuclear plants, especially smaller plants based on modular designs. Nuclear plants can run virtually continuously, typically at capacity factors of 90%, obviating the need for battery storage. Supplying 635 TWh of electricity for all ZEVs would require building about 80 new 1,000-MW nuclear plants by 2050, or a larger number of smaller, modular units.[154]
The Value of CO2 Emissions Reductions
Proponents of ZEV subsidies often emphasize the benefits of the accompanying reductions in CO2 emissions. As discussed previously, EIA’s projected stock of ZEVs will reduce U.S. CO2 emissions by a total of 867 million tons over 2018–50, versus CO2 emissions from the same number of ICVs. Over this same period, EIA forecasts total energy-related CO2 emissions of about 168 billion tons.[155] Thus, an equivalent number of ICVs would increase U.S. energy-related CO2 emissions by about just half of 1%.
By comparison, EPA estimated that its Clean Power Plan (CPP), which the current administration proposes to repeal, would reduce CO2 emissions by more than 400 million tons annually by 2030.[156] Based on EPA’s estimates through that year, the projected CO2 emissions reductions would exceed 1 billion tons/year by 2050.[157] CPP’s cumulative emissions reductions relative to EPA’s business-as-usual scenario would be about 18.4 billion tons from 2018 through 2050, roughly 22 times larger than the reduction of 896 million tons associated with ZEVs versus ICVs (Figure 17). Even if all EVs were powered solely by renewable energy, by 2050, the resulting CO2 emissions reductions would be less than 500 million tons/year, half the annual projected reduction under the CPP.
In EPA’s Regulatory Impact Analysis report on CPP, the agency never discussed whether CPP would have any measurable impact on world climate. But independent analyses using the EPA-sponsored Model for the Assessment of Greenhouse Gas Induced Climate Change (MAGICC) estimated that CPP would reduce the world’s average temperature only 0.004°C–0.013°C by 2100, with an average reduction of 0.008°C.[158] Changes in average global temperature of those magnitudes are far too small to be measured physically. Nor can such small changes be separated from natural climate variation. Thus, the projected reductions in CO2 emissions under CPP would have no measurable impact on world climate, and thus no actual benefits.[159] Given the far smaller magnitude of the estimated CO2 reductions associated with EIA’s projected increase in ZEV ownership, those emissions reductions also would have no measurable climate impacts and therefore no climate benefits.[160]
V. Additional Costs and Equity Impacts of ZEV Subsidies
ZEV subsidies and mandates will not only worsen air pollution levels relative to ICVs; they have additional social costs, including increased inequity. In effect, ZEV subsidies and mandates now allow affluent ZEV owners to capture benefits for themselves while socializing the costs to everyone else, especially lower-income consumers.
For example, preferred HOV access for ZEV owners is an especially pernicious subsidy because it reduces the time savings for others who use the lanes. In other words, ZEV owners impose a direct external cost on other HOV users. In fact, previous research has shown that allowing single-occupant vehicles to use HOV lanes exacerbates congestion costs for carpoolers and far outweighs any environmental benefits from increased use of ZEVs.[161] The more troubling equity impact is that ZEV subsidies unfairly burden poorer individuals; while most ZEVs are purchased by wealthier individuals, the costs are borne by the former, along with businesses.
In addition to the 2013 study previously mentioned that found Tesla owners had average household incomes of $293,200, another 2013 study by researchers at the University of California–Davis found that 96% of California ZEV owners live in single-family homes and 83% had household incomes above $100,000, including 46% with household incomes above $150,000.[162] By comparison, according to the U.S. Census Bureau, 2013 median household income in California was $60,190, and fewer than 15% of all households had incomes above $150,000.[163] A nationwide study by Experian Automotive found that nearly 21% of all ZEV buyers in 2013 had household incomes greater than $175,000.[164] And a nationwide survey of PHEV and BEV owners in 2017 found that 56% had household incomes of at least $100,000 and 17% had household incomes of at least $200,000.[165]
As more mid-priced ZEVs are brought to market (as opposed to high-end vehicles such as the Tesla Model S), their average prices should decrease, which will encourage greater adoption of ZEVs in the mass market. This will also reduce the household income disparity between ZEV purchasers and others. Nevertheless, ZEV subsidies paid to owners up to this point, such as the federal government’s income tax credit of up to $7,500 per vehicle and California’s Clean Vehicle program rebates of up to $5,000 per vehicle, primarily have benefited higher-income consumers. Moreover, because the federal tax credit starts to expire for each manufacturer after it sells 200,000 ZEVs, consumers with lower household incomes who want to buy at that point may be unable to obtain the credit. Similarly, many existing subsidies described previously will expire in the next few years. As these subsidies expire, the economic incentives to purchase ZEVs will similarly decrease.
The development of a ZEV charging infrastructure will also benefit higher-income consumers, for several reasons. First, residential charging systems are primarily installed in single-family buildings by homeowners with above-average incomes. Although charging systems for apartments and condominiums are becoming available, residents do not receive direct subsidies, as homeowners do. Second, charging system costs, along with needed investments in distribution system infrastructure, are allocated by electric utility regulators based on traditional methods that spread costs widely across all customers. For example, in California, the costs of PG&E’s $160 million program to install 7,500 Level 2 chargers and 100 DCFCs will be allocated among residential, commercial, and industrial customers, with about 50% of the costs being allocated to residential customers.
The electric distribution infrastructure includes the utility distribution network of poles and wires, as well as the electric meters equipment and the charging equipment installed at a customer’s location (Figure 18).
The distribution network (the left side of Figure 18) must be sized to meet the peak electricity demand of its users. As more customers purchase ZEVs and install charging equipment, and as increased battery capacities require higher-voltage chargers, peak demand will most likely increase.
If electric utilities must upgrade their distribution service to accommodate additional BEV charging, they will allocate the costs among all customer groups using standard cost-allocation procedures. Although these procedures are generally based on cost-causation—those who cause the costs to be incurred also pay those costs—these procedures rarely attribute costs to actions of specific customers.[166] Thus, distribution infrastructure upgrades are typically allocated to all customers—residential, commercial, and industrial—without singling out ZEV users. Instead, the costs are added to everyone’s bills, either in the form of a higher minimum bill charge[167] or higher per-kWh rates.
In large measure, this type of pricing occurs because local distribution systems provide nonexclusive service; along a distribution network, it is not possible to exclude individual customers from electric service (unless, of course, their service is canceled for nonpayment). Thus, if a utility upgrades a distribution circuit to accommodate larger peak demand because a few customers have purchased ZEVs, the costs of the upgrade are allocated to all residential customers, not just the ZEV purchasers.[168] Again, ZEV owners enjoy private benefits, while many of the costs they cause are socialized.
By contrast, the 156,000 gasoline stations in the U.S., which are the refueling infrastructure for ICVs, were not paid for with public funds and are supported only by their customers. Thus, the private costs are not socialized.
Yet another equity issue arises because of the incentives for ZEV owners to install behind-the-meter solar photovoltaic (PV) systems to charge their vehicles. Some advocates claim: “Solar panels and electric cars are a match made in heaven—when you install a solar energy system on your home, you can use it to both power your home and charge your electric car for emissions-free transportation.”[169] But behind-the-meter solar PV subsidies worsen the inequities of subsidies for ZEV chargers. This is especially true with “net-metering” programs in which residential and commercial retail customers “sell” the solar electricity they generate back to their local utility at above-market rates.
The costs to the electric utility from purchasing solar power at above-market rates must be recovered from all other customers. For example, if the current average wholesale price of electricity is 5 cents per kWh, but a utility pays a customer for solar power at the utility’s retail rate of 15 cents per kWh, the additional 10 cents per kWh must be recovered from other customers.
As discussed previously, solar power is inherently intermittent. Therefore, most customers who install behind-the-meter solar PV remain connected to the local distribution grid. As such, their local utility provides backup service to them at little or no cost. The reason is that typical utility minimum bill charges are set too low to recover all the fixed costs of providing service. The remaining fixed costs, including the costs of backup generating capacity, are incorporated into the per-kWh charges on utility bills.
Some net-metering programs allow residential and business customers to avoid the minimum bill payments and instead receive rebates from their local utility. In those instances, the customer does not pay for any of the fixed costs or the cost of backup service.
Furthermore, increasing solar PV along a distribution circuit creates greater voltage instability, as when the sun goes behind a cloud and then reappears. To combat that instability, which could otherwise lead to service failures, utilities must install specialized equipment. (It also means that the amount of PV along a given circuit may be limited to a relatively small percentage of a circuit’s capacity. A typical rule of thumb is 15%,[170] although some circuits can be configured to accept percentages above 30%.)[171]
Behind-the-meter thus imposes costs on all customers of an electric utility, but the benefits primarily redound to the wealthier ones. Even with subsidies, behind-the-meter solar PV installations are expensive. For example, the true cost of a typical residential solar PV installation—excluding the current 30% federal tax credit that will be phased out starting in 2019—is between $15,000 and $30,000, depending on the size of the system installed.[172] Lower-income individuals and those who dwell in apartments and condominiums are unlikely to benefit from behind-the-meter solar PV but must bear the additional costs imposed by solar PV owners on the local utility.
Thus, ZEV owners, who have higher income than average and are far more likely to own a single-family home, receive subsidies for their vehicles and home charging systems; can use HOV lanes to the detriment of other drivers; can use public charging stations whose installation is often subsidized; and can install subsidized behind-the-meter solar PV systems with which to recharge their ZEVs at home. Lower-income individuals who cannot afford ZEVs, as well as businesses, must pay a disproportionate share of these subsidies through higher taxes and higher electricity rates.
VI. Conclusion
The newest ZEVs are impressive technologically. But there is no economic basis for the billions of dollars spent subsidizing their adoption. The entire premise for subsidizing ZEVs and the infrastructure needed to power them—reduced air pollution and lower CO2 emissions—is flawed.
The simple fact is that, because of stringent emissions standards and low-sulfur gasoline, new ICVs today emit very little pollution, and they will emit even less in the future. Compared with new ICVs, ZEVs charged with the forecast mix of electric generation will emit more criteria air pollutants—SO2, NOx, and particulates—not less. And although ZEVs will emit less CO2 than ICVs, the projected reduction in CO2 emissions, below 1% of total forecast U.S. CO2 emissions, will have no measurable impact on climate and, hence, no economic value.
ZEV subsidies also impose disproportionate costs on lower-income consumers to benefit higher-income ones. Historically, ZEV purchasers have had much higher household incomes than average. Moreover, ZEV purchasers are primarily homeowners, who benefit not only from subsidies to purchase their vehicles but also from subsidies to install charging and solar PV systems.
ZEV purchasers who install behind-the-meter solar PV reap additional subsidies by not paying the full costs of providing them with backup power, not paying the full costs for upgrading local electric utility distribution systems to support their ZEVs, and not paying the full costs of utility-owned public charging stations that they can use.
To be sure, at a local level—e.g., a crowded downtown—air quality would likely improve if all existing ICVs were replaced today with ZEVs. But similar improvements in air quality also would be realized by replacing existing ICVs with new ICVs, because new ICVs emit very little pollution. Moreover, depending on where air pollution emitted by electric-generating plants disperses, local air quality in urban areas could decrease with additional ZEVs. Finally, local variations in electricity sources and the times at which ZEVs are charged can change the relative emissions of ZEVs and ICVs. For example, a ZEV that is charged when electricity demand is highest is likely to be charged with electricity from less efficient and higher-emissions generating resources, such as oil-fired peaking plants.
Absent continued subsidies that significantly reduce the costs of ZEVs and charging infrastructure, breakthroughs in battery technology that are commercialized successfully, or bans on ICV sales, there appears to be little likelihood that ZEVs will—or should—replace a large fraction of ICVs in the U.S. for the foreseeable future.
The bottom line is that the economic and environmental rationales for subsidizing ZEVs do not withstand scrutiny. These subsidies, along with mandates for ZEV adoption, should be eliminated.
Endnotes
- Executive Order B-48-18, Jan. 26, 2018.
- See, e.g., Jeffrey Sachs, “Electric Cars Are Driving the Transition to Sustainable Technologies,” The Guardian, Sept. 21, 2009.
- “The Death of the Internal Combustion Engine,” The Economist, Aug. 12, 2017.
- Peter Holley, “Why 2017 Will Go Down as the Beginning of the End of the Internal Combustion Engine,” Washington Post, Oct. 11, 2017.
- Jim Holder, “Internal Combustion Engine to Be Dead by 2050, Says Toyota R&D Boss,” Autocar, Nov. 13, 2017.
- Norman Mayerson, “The Internal Combustion Engine Is Not Dead Yet,” New York Times, Aug. 17, 2017.
- Chris Perkins, “Mazda Says Its Next-Generation Gasoline Engine Will Run Cleaner than an Electric Car,” Road and Track, Jan. 29, 2018.
- Sean Szymkowski, “UK to Ban Diesel, Gasoline Car Sales by 2040; Follows France, Norway, Holland Bans,” Green Car Reports, July 27, 2017.
- Jackie Wattles, “India to Sell Only Electric Cars by 2030,” CNN Tech, June 3, 2017.
- In 2016, the German Bundestag passed a nonbinding resolution calling for Europe to ban internal combustion engines by 2030. See Jonathan Gitlin, “Germany’s Bundesrat Votes to Ban the Internal Combustion Engine by 2030,” ArsTechnica, Oct. 10, 2016.
- Chris Morris, “A California Lawmaker Is Trying to Ban All Gasoline-Powered Cars in the State by 2040,” Fortune, Dec. 6, 2017. The legislation is: AB-1745 Vehicles: Clean Cars 2040 Act. It would cover both gasoline- and diesel-powered vehicles.
- ZEVs encompass three categories of vehicles: (i) plug-in hybrid vehicles (PHEVs), which have both an internal combustion engine and batteries; (ii) purely battery-powered vehicles (BEVs); and (iii) vehicles that use hydrogen fuel cells (FCVs) to produce electricity.
- Executive Order B-48-18, Jan. 26, 2018.
- Executive Order B-16-12, Mar. 23, 2012.
- Those states are Connecticut, Maine, Maryland, Massachusetts, New Jersey, New York, Oregon, Rhode Island, and Vermont.
- California Air Resources Board, “Zero-Emission Vehicle Standards for 2018 and Subsequent Model Year Passenger Cars, Light-Duty Trucks, and Medium-Duty Vehicles,” CA CCR 1962.2.
- Tesla, Inc., 2017 Form 10-K, p. 72. Tesla also reported tax credits of $208 million associated with its construction of a battery-manufacturing facility in Nevada.
- Executive Order B-48-18.
- For an introduction, see Michael Katz and Carl Shapiro, “Network Externalities, Competition, and Compatibility,” American Economic Review 75, no. 3 (June 1985): 424–40.
- Chris Ross, “Robin Hood Rides Again: Lifting the Electric Vehicle Tax Credit,” Forbes, Nov. 14, 2017.
- Daniel Gross, “Green Privilege,” Slate, June 24, 2015.
- Eric Loveday, “Strategic Vision Says Testosterone Is What Sells the Tesla Model S to Wealthy Americans,” InsideEVs, Sept. 6, 2013.
- Christopher DeMoro, “EV Buyers Are Younger, Richer, and Looking for a Deal,” EV Obsession, May 6, 2015.
- Federal Reserve Bank of St. Louis, FRED Database, Median Household Income in the United States.
- Evelyn Chang, “Tesla Shares Fall After Moody’s Downgrades Credit Rating,” CNBC, Mar. 27, 2018.
- Fred Lambert, “Tesla Model 3 Production Aims for 6,000 Units per Week in June After Upgrade in May—5,000 with Margin of Error, Says Elon Musk,” electrek, Apr. 17, 2018.
- Auto Alliance, “Advanced Technology Vehicle Sales Dashboard,” 2018. This figure includes sales to state and local governments.
- Ibid. and U.S. Bureau of Economic Analysis, “Motor Vehicle Unit Retail Sales,” Mar. 29, 2018. Different sources report different ZEV sales numbers. For example, InsideEVs reports slightly higher sales for all ZEVs than does Auto Alliance but does not separate U.S. sales for Tesla from overseas sales. (Tesla does not publish a breakdown of its sales by country.) For consistency, this report relies on the U.S. registration data published by Auto Alliance.
- Research and Markets, “Hydrogen Fuel Cell Vehicles—A Global Analysis,” March 2018.
- Auto Alliance, “Advanced Technology Vehicle Sales Dashboard,” 2018.
- Toyota Motor Co., “Hydrogen Is Going the Distance,” Jan. 23, 2018. Two other automakers manufacture FCVs: Honda, which leases, but does not sell, its Clarity FCV; and Hyundai, which also leases, but does not sell, an FCV of its Tucson model. Hyundai has announced that it will offer a new FCV, called the Nexo, by the end of 2018.
- Eric Loveday, “Supercars Beware: New Tesla Roadster Compared to Bugatti Chiron,” InsideEVs, Nov. 20, 2017.
- EIA, Annual Energy Outlook 2018, Feb. 6, 2018, table 40. This calculation excludes natural gas–powered vehicles and “flex-fuel” vehicles that can burn fuel that is up to 85% ethanol.
- U.S. Internal Revenue Service, “Plug-In Electric Vehicle Credit IRC-30D.”
- Known colloquially as “carpool” lanes.
- California Air Resources Board, “Clean Vehicle Rebate Project,” 2018.
- Los Angeles Dept. of Water and Power, Electric Vehicle Incentives.
- For a discussion of the effects of subsidies in Europe, see Petra Zsuzsa-Lévay, Yannis Drossinos, and Christian Thiel, “The Effect of Fiscal Incentives on Market Penetration of Electric Vehicles: A Pairwise Comparison of Total Cost of Ownership,” Energy Policy 105 (June 2017): 524–33.
- Edmunds, “Elimination of Federal Tax Credits Likely to Kill U.S. EV Market,” April 2017.
- U.S. Environmental Protection Agency (EPA), “EPA and NHTSA Finalize Historic National Program to Reduce Greenhouse Gases and Improve Fuel Economy for Cars and Trucks,” April 2010, p. 4.
- U.S. Dept. of Energy (DOE), Office of Energy Efficiency and Renewable Energy, “Electric Vehicles: Tax Credits and Other Incentives.”
- Rhode Island offered purchase rebates until the end of 2017, when funding ran out.
- See, e.g., State of California, Governor’s Interagency Working Group on Zero-Emissions Vehicles, 2016 ZEV Action Plan, October 2016.
- California is also requiring German automaker Volkswagen AG to spend $800 million on charging stations over the next 10 years as part of the state’s settlement with the company over the diesel emissions scandal. See California Air Resources Board, “CARB Approves $200 Million VW Zero-Emission Vehicle Investment in California,” July 27, 2017.
- This topic is discussed below.
- As discussed below, several states have begun to levy special registration fees to compensate for the loss of gasoline taxes.
- A quirk of ZEV batteries is that not all of their rated capacity can be used. For example, the BMW i3 has a battery capacity of 33 kWh, but the battery cannot discharge the full 33 kWh because it operates more efficiently if never allowed to charge or discharge fully.
- InsideEVs, “Monthly Plug-In Sales Scorecard.” The InsideEVs reported sales estimate for Tesla is 162,000, which includes overseas sales. The individual manufacturer sales data have been adjusted by this analysis to reflect the 722,000 U.S. registrations for all ZEVs, as reported by Auto Alliance.
- InsideEVs, “US Federal $7,500 Electric Vehicle Credit Expiry Date by Automaker.”
- The state paid just over $13 million in rebates for fuel-cell vehicles. See Clean Vehicle Rebate Project, “CVRB Rebate Statistics,” Mar. 13, 2018.
- The $5,000 maximum rebate applies to fuel-cell vehicles. The maximum rebate for BEVs and PHEVs is $2,500.
- An additional $15 million is earmarked for consumer awareness programs and subsidies for public charging stations.
- HomeAdvisor, “How Much Do Electric Vehicle Charging Stations Cost to Install?” September 2017.
- Idaho National Laboratory, “Plugged In: How Americans Charge Their Electric Vehicles,” INL/EXT-15-35584, 2015, p. 18.
- Calculated as: (517,000 ZEVs) x ($1,000 installation cost) x (30% tax credit) x (100% of owners installing).
- See Suzanne Guinn, “EVSE Rebates and Tax Credits, by State,” ClipperCreek.com, Oct. 10, 2017, for a complete list of ZEV charging station rebates.
- ClipperCreek, “How Long Does It Take to Charge an Electric Car?” Feb. 5, 2018.
- A DCFC operates at 480 volts or higher and a power output of between 50 kW and 120 kW, larger than the power input into an entire home. (For example, a house with a 200-amp service meter at 240 volts has a maximum capacity of (200 A) x (240 V) = 48 kW.
- Eric Taub, “For Electric Car Owners, ‘Range Anxiety’ Gives Way to ‘Charging Time Trauma,’ ” New York Times, Oct. 17, 2017.
- See, e.g., Steve Dent, “Samsung’s ‘Graphene Ball’ Battery Could Lead to Fast-Charging EVs,” Engadget, Nov. 29, 2017.
- The equity ramifications of allocating charging station costs among all utility ratepayers are discussed in Section V. A discussion of the regulatory methods by which utility costs are allocated among ratepayer groups can be found in Jonathan A. Lesser and Leonardo R. Giacchino, Fundamentals of Energy Regulation, 2d ed. (Reston, Va.: Public Utility Reports, Inc., 2013), chap. 6.
- In the Matter of the Application of Pacific Gas and Electric Company for Approval of Its Electric Vehicle Infrastructure and Education Program, Decision 16-12-065, Dec. 15, 2016. The agreement reflected in this order does not provide data on the average cost per charging port or the number of charging ports per charging station.
- City of New York, “Leading the Charge: Mayor Announces Fast-Charging EV Hubs in All 5 Boroughs,” Sept. 20, 2017.
- Southern California Edison, “Charge Ready Pilot Program Quarterly Report for Fourth Quarter 2017,” Mar. 1, 2018, p. A-14.
- Austin Energy FOIA Response.
- Ethan N. Elkind, “Plugging Away: How to Boost Electric Vehicle Charging Infrastructure,” UC Berkeley School of Law, Center for Law, Energy & the Environment, June 2017.
- Abdulkadir Bedir et al., “California Plug-In Electric Vehicle Infrastructure Projections: 2017–2025,” CEC Staff Report, March 2018.
- Ibid., p. 9.
- DOE, Alternative Fuels Center.
- Ibid., “Public Retail Gasoline Stations by State and Year,” 1996–2012.
- Eric Wood et al., “National Plug-In Electric Vehicle Infrastructure Analysis,” DOE, Office of Energy Efficiency and Renewable Energy, September 2017, pp. vi–vii.
- DataUSA: San Francisco, CA, Housing and Living.
- Ibid., Los Angeles, CA, Housing and Living.
- Ibid., New York, NY, Housing and Living.
- Los Angeles Department of Water and Power, “Drive Electric,” 2013.
- County of Sonoma, California, “Electric Vehicle Charging Station Program and Installation Guidelines,” July 2011.
- State of Nevada, Office of Energy, “Nevada Electric Highway.”
- Kristy Hartman and Emily Dowd, “State Efforts to Promote Hybrid and Electric Vehicles, National Council of State Legislatures,” Sept. 26, 2017.
- Lauren Sommer, “California Lawmakers Allow Clean Cars to Stay in Carpool Lanes,” KQED, Sept. 15, 2017.
- Ibid.
- Carlos Carrion and David Levinson, “Value of Travel Time Reliability: A Review of Current Evidence,” Transportation Research Part A: Policy and Practice 46, no. 4 (May 2012): 720–41.
- Sharon Shewmake and Lovell Jarvis, “Hybrid Cars and HOV Lanes,” Transportation Research Part A: Policy and Practice 67, issue C (September 2014): 304–19.
- Julian Spector, “Updated: 17 States Now Charge Fees for Electric Vehicles,” Greentech Media, July 5, 2017.
- Minnesota Statute 168.013.
- California Road Repair and Accountability Act of 2017, sec. 47.
- “The Death of the Internal Combustion Engine,” The Economist.
- Some argue that ZEVs are economical, but “barriers to entry” prevent their adoption without subsidies and mandates. Such arguments fundamentally misconstrue the nature of entry barriers. Entry barriers are defined as costs imposed on new entrants that are not similarly imposed on incumbents, or benefits provided to incumbents not provided to new entrants. For a discussion, see George Stigler, The Organization of Industry (Homewood, Ill.: Irwin, 1968).
- Below, the report addresses CO2 emissions, which are not “criteria” air pollutants under the U.S. Clean Air Act.
- Perhaps the most comprehensive analysis of vehicle costs and benefits is Victoria Transport Policy Institute, Transportation Cost and Benefit Analysis Techniques, Estimates and Implications, 2d ed. (October 2016) (VTI C-B Study 2016). A more limited example comparing ownership and externality costs is Lambros Mitropoulos, Panos Prevedouros, and Pantelis Kopelias, “Total Cost of Ownership and Externalities of Conventional, Hybrid and Electric Vehicles,” Transportation Research Procedia 24 (2017): 267–74.
- VTI C-B Study 2016, Chapter 5.10, p. 5-10.27, table 5.10.7-1.
- Jason Lemp and Kara Kockelman, “Quantifying the External Costs of Vehicle Use: Evidence from America’s Top Selling Light-Duty Models,” Transportation Research Part D: Energy and the Environment 13 (December 2008): 491–504.
- For a summary discussion, see Richard Lattanzio and James McCarthy, “Tier 3 Motor Vehicle Emission and Fuel Standards,” Congressional Research Service, Apr. 28, 2014.
- Id., pp. 4–5.
- A 2016 study compared the emissions from an electric Ford Focus with its gasoline-powered equivalent based on geographic location and marginal generating resources. See Stephen Holland et al., “Are There Environmental Benefits from Driving Electric Vehicles? The Importance of Local Factors,” American Economic Review 106, no. 12 (December 2016): 3700–3729.
- EIA Annual Energy Outlook 2018, table 8.
- PHEVs use both electricity and gasoline. For shorter trips (e.g., commuting to work), they can rely exclusively on electricity. However, data are not available that would allow one to forecast the relative use of electricity and gasoline for hybrids over time. Presumably, as battery technology improves, allowing PHEVs to travel farther on battery power, they will rely less on their internal combustion engines. For purposes of this analysis, therefore, we assume 100% electricity consumption for ZEVs.
- EIA Annual Energy Outlook 2018, table 7.
- Internal combustion engine range can also decrease in extreme cold and heat. In contrast to ZEVs, however, excess heat from the engine is used to heat the interior in the winter.
- Tugce Yuksel and Jeremy Michalek, “Effects of Regional Temperature on Electric Vehicle Efficiency, Range, and Emissions in the United States,” Environmental Science & Technology 49, no. 6 (February 2015): 3974–80.
- An S-curve is generally based on a logistic function with this form:
, where %VT = the percentage of vintage V remaining after T years, L = the average lifetime, and k is a shape parameter. Here, k = 0.40. The higher the value of k, the slower the initial retirement rate of the vehicle. Using this specification, for example, with a 10-year average life, after 5 years, about 8% of the original vintage vehicles will have been scrapped. After 8 years, 27% will have been scrapped, and so forth.
- Given the assumption about improving energy efficiency, the lower the assumed average life value, the more quickly the average overall energy efficiency of the ZEV stock will increase and, hence, the lower will be generation-related emissions.
- Mathematically, the calculation is:
, where kWh2030 = total electric energy consumption of ZEVs in the year 2030; EVt = the number of new ZEVs in year t; %Vt, 2030 = the percent of new ZEVs of vintage t remaining in the year 2030; and et = the average energy efficiency of new ZEVs of vintage t.
- U.S. EIA, Frequently Asked Questions, “How Much Electricity Is Lost in Transmission and Distribution in the United States?” Jan. 29, 2018.
- EIA Annual Energy Outlook 2018, table 8.
- Although coal-fired power plants emit mercury, EPA’s estimates of total damages from mercury emissions are small. Thus, for the purposes of this analysis, I have excluded mercury emissions.
- EIA Annual Energy Outlook 2018, table 8.
- Ibid.
- The math is as follows. For SO2: (4,198 TWh) x (109 kWh/TWh) / (1.25 x 106 tons) / (2000 lbs/ton) = 0.0006 lbs/kWh. The NOx calculation is similar.
- Yu-Fei Xing et al., “The Impact of PM2.5 on the Human Respiratory System,” Journal of Thoracic Disease 8, no. 1 (January 2016): E69–E74.
- Particulate emissions from oil-fired generating plants totaled about 15,000 tons in 2014: see EPA, 2014 Emissions Inventory. Because oil-fired generation (both petroleum liquids and petroleum coke) has decreased steadily in the last 10 years and is expected to decline further, I ignore the contribution of particulates from oil-fired generation. For example, in 2016, oil-fired totaled about 24,000 GWh, less than 1% as much as coal and natural gas–fired generation of about 2.6 million GWh. See EIA Electric Power Annual 2016, table 3.1.
- One GWh = 1 million kWh.
- DOE, National Technical Energy Laboratory, “Cost and Performance Baseline for Fossil Energy Plants, Volume 1a: Bituminous Coal (PC) and Natural Gas to Electricity,” revision 3, July 6, 2015, p. 29, exhibit 2-6.
- Although some studies have estimated “life-cycle” emissions of different vehicles (emissions associated with manufacturing vehicles and batteries, extracting and transporting coal and natural gas, scrapping and disposing of vehicles at the end of their useful life, etc.), this analysis focuses solely on direct emissions. For an example of a life-cycle analysis, see Troy Hawkins et al., “Comparative Life Cycle Assessment of Conventional and Electric Vehicles,” Journal of Industrial Ecology 17, no. 1 (2012): 53–64.
- Two grams of SO2 are produced for every gram of sulfur (S), as the molecular weight of SO2 is 64 and that for S is 32. Therefore, one pound of S produces two pounds, or 908 gm, of SO2. A gallon of gasoline weighs about 6.07 pounds. Ten ppm is equivalent to (10/106). Finally, when gasoline is burned, a small percentage, about 3%, is converted to SO4. Thus, the amount of SO2 emitted per gallon of gasoline is: (908 gm SO2/lb-S) x (6.07 lbs/gallon) x (10/106) x (1 – 0.03) = 0.0535 gms-SO2/gallon.
- As discussed below, on Apr. 3, 2018, EPA Administrator Scott Pruitt announced that the agency was going to revise the existing GHG emissions standards for cars and light trucks for 2022–25. The GHG standards are translated into CAFE standards. The agency will be working with the National Highway Traffic Safety Commission to issue a Notice of Proposed Rulemaking that will take comments. As of this writing, no date has been set for issuance of revised standards, and it is not known what those revised standards will be.
- Scott Vaughan, “Average Lifespan of U.S. Vehicles.” See also Antonio Bento, Kevin Roth, and Yiou Zuo, “Vehicle Lifetime Trends and Scrappage Behavior in the U.S. Used Car Market,” The Energy Journal 39, no. 1 (January 2018): 159–84. They estimate that the average lifetime of a U.S. passenger car is 15.6 years. The average lifetime is a function of both operational and economic factors, including the cost of new replacement vehicles.
- Even if the combined CAFE standards for cars and light trucks remain constant at their 2021 level, total SO2 emissions (vehicles and refineries) will still be far less than those for ZEVs.
- If the CAFE standards are assumed to remain constant at their 2021 level through 2050, PM emissions associated with refineries would increase slightly because total gasoline consumption for ICVs would increase. The cumulative difference in PM emissions over the 2016–50 period would increase to about 22,000 tons.
- Blended gasoline is so named because it is mixed with 10% ethanol.
- EPA, “Final Rule for Model Year 2012–2016 Light-Duty Vehicle Greenhouse Gas Emission Standards and Corporate Average Fuel Economy Standards,” 2010.
- EPA, “Final Rule for Model Year 2017 and Later Light-Duty Vehicle Greenhouse Gas Emissions and Corporate Average Fuel Economy Standards,” 2012.
- A summary of the annual emissions standards, which also depend on the size of vehicles (called the vehicle “footprint”), can be found in EPA’s 2012 Regulatory Announcement.
- EPA, “EPA Administrator Pruitt: GHG Emissions Standards for Cars and Light Trucks Should Be Revised,” Apr. 2, 2018.
- In 2009, the Obama administration granted a waiver to California to set its own fuel economy standards. This has been controversial because it can require automakers to meet two different fuel efficiency standards, thus increasing costs. EPA Administrator Pruitt has stated that he wishes to maintain one standard for the entire country.
- EIA Annual Energy Outlook 2018, table 18. EIA forecast is 178 billion metric tonnes of CO2, equivalent to (178) x (1.10) = 196 billion short tons.
- For example, EPA’s Regulatory Impact Analysis of the Clean Power Plan assumed that anyone who dies from heart or lung disease, regardless of age, suffers a “premature” death. For a discussion, see Jonathan Lesser, “Missing Benefits, Hidden Costs: The Cloudy Numbers in the EPA’s Proposed Clean Power Plan,” Manhattan Institute, June 2016, pp. 27–28.
- National Research Council, Hidden Costs of Energy: Unpriced Consequences of Energy Production and Use (Washington, D.C.: National Academies Press, 2010), pp. 82–90.
- Federal Reserve Bank of St. Louis, FRED (Economic Research), “Gross Domestic Product: Implicit Price Deflator.”
- Projecting the 2014 average emissions rates for particulates reported in EPA emissions inventory, together with EIA’s forecast of coal-fired and natural gas–fired generation through 2050, the resulting PM10 and PM2.5 damages would be $137 million in 2050.
- If EPA mileage standards are assumed to remain at their 2021 levels through 2050, total NOx and SO2 damages would increase slightly, with annual damage increasing by about 10%, to just over $55 million in 2050. The increase in NOx emissions is associated with refinery output of additional gasoline to fuel lower-mileage IC vehicles, while the increase in SO2 emissions reflects both an increase in refinery emissions and an increase in vehicle emissions.
- The present values are as of 2018 and assume a 3% social discount rate, which is a typical value used by government agencies, including EPA. If the 2021 mileage standards are assumed to remain unchanged through 2050, the present value of ICV damages increases to about $872 million.
- EIA Annual Energy Outlook 2018, table 55. In 2050, EIA projects total renewable generation of 1,651 TWh from all sources and total generation of 5,396 TWh.
- Despite having no CO2 emissions, nuclear power is typically not considered as an alternative by those advocating for an emissions- and carbon-free electricity mix. This makes little sense, especially as baseload nuclear power avoids the need for backup storage and generation for intermittent wind and solar power.
- California Renewables Portfolio Standard; New York State Clean Energy Standard.
- EIA, Electricity Power Monthly, February 2018, table 5.6.B.
- See North Carolina Clean Energy Technology Center, Database of State Incentives for Renewables & Efficiency, July 2017.
- Galen Barbose, “U.S. Renewables Portfolio Standards: 2017 Annual Status Report,” Lawrence Berkeley National Laboratory, LBNL-2001031, July 2017, p. 6.
- EIA, Electric Power Monthly, February 2018, table 5.6.B.
- For a discussion of adoption of new technologies, see Everett Rogers, Diffusion on Innovations, 5th ed. (New York: Simon & Schuster, 2003).
- Mark Z. Jacobson et al., “Low-Cost Solution to the Grid Reliability Problem with 100% Penetration of Intermittent Wind, Water, and Solar for All Purposes,” Proceedings of the National Academy of Sciences 112, no. 49 (Dec. 8, 2015): 15060–65.
- Christopher T. M. Clack et al., “Evaluation of a Proposal for Reliable Low-Cost Grid Power with 100% Wind, Water, and Solar,” Proceedings of the National Academy of Sciences 114, no. 26 (June 27, 2017): 6722–27.
- Matthew Shaner et al., “Geophysical Constraints on the Reliability of Solar and Wind Power in the United States,” Energy and Environmental Science 11, no. 4 (February 2018): 914–25.
- The math is as follows: in 2050, EIA projects 3.376 trillion total light-duty VMT. If all light-duty vehicles were BEVs, then assuming average electricity use of 0.20 kWh/mile and 5% transmission and distribution losses, total ZEV electricity consumption would be (3.376 x 1012 miles) x (0.20 kWh/mile) x (1.05) / (1012 kWh/TWh) = 709 TWh.
- EIA Annual Energy Outlook 2018, table 3.2, shows 2016 electricity consumption of 3,917 TWh. Thus, EIA’s projected increase in consumption is 4,708 TWh – 3,917 TWh = 791 TWh, compared with the 635 TWh increase in electricity consumption if all vehicles are ZEVs in 2050.
- EIA, Electric Power Monthly, January 2018, table 6.7B.
- The math is as follows: capacity = (635,000 MWh) / (8,760 hours/year) / 0.3 = 241,630 MW.
- This figure is based on an average wind power density of 3 watts per square meter. See Paul Denhold et al., “Land-Use Requirements of Modern Wind Power Plants in the United States,” National Renewable Energy Laboratory, NREL/TP-6A2-4583, August 2009, p. 14.
- American Wind Energy Association, “Wind Energy Facts at a Glance.”
- Energy.gov, “Renewable Electricity Production Tax Credit (PTC).”
- Robert Bryce, “Wind Power Is an Attack on Rural America,” Los Angeles Times, Feb. 27, 2017. See also idem, “Wind Power Hits Headwinds in New York,” Albany Times-Union, Sept. 27, 2017. As Bryce points out, opposition to offshore wind power is also increasing.
- EIA, Electric Power Monthly, January 2018, table 6.7B.
- Based on data from a battery storage facility built by Southern California Edison. In 2017, the average generation provided per MW of battery storage is 4 MWh. See Megan Guess, “A Look at the New Battery Storage Facility in California Built with Tesla Powerpacks,” ArsTechnica, Jan. 31, 2017.
- The cost of the unit itself is $5,500. The estimated cost of supporting hardware is $700. The estimated range of installation costs is $800 to $2,000. See Andy Seridy, “Pegging the All-In, Installed Cost of a Tesla Powerwall 2,” SolarReviews, Oct. 3, 2017.
- For a discussion of small nuclear reactor technology, development, and costs, see World Nuclear Association, “Small Nuclear Reactors,” March 2018.
- EIA Annual Energy Outlook 2018, table 18.
- EPA, “Regulatory Impact Analysis for the Clean Power Plan Final Rule,” August 2015, p. ES-6, table ES-4.
- To do this, the analysis projects the same rate of CO2 emissions reductions from 2020 to 2030 through the year 2050.
- Bjorn Lomborg, “Impacts of Current Climate Proposals,” Global Policy 7, no. 1 (February 2016): 109–18. To develop these temperature-change estimates, Lomborg uses MAGICC, which was funded by EPA and developed by the National Center for Atmospheric Research. A description of MAGICC can be found at https://www.cgd.ucar.edu/cas/wigley/magicc. The model can be downloaded at https://www.cgd.ucar.edu/cgi-bin/cas/magicc.cgi. See also testimony of Paul Knappenberger before the U.S. House of Representatives Committee on Science, Space, and Technology, “The Administration’s Empty Promises for the International Climate Treaty,” Nov. 18, 2015. Knappenberger also uses MAGICC to project temperature changes resulting from CPP and the administration’s stated goal of an 80% reduction in CO2 emissions by 2050. Using MAGICC, he estimates that the 80% reduction will yield a global temperature reduction of 0.11°C.
- For a detailed discussion, see Lesser, “Missing Benefits, Hidden Costs,” pp. 18–19.
- This argument does not consider the additional empirical flaws in estimates of the “social cost of carbon” itself. A discussion of these flaws can be found in ibid., pp. 9–16.
- Antonio Bento et al., “The Effects of Regulation in the Presence of Multiple Unpriced Externalities: Evidence from the Transportation Sector,” American Economic Journal: Economic Policy 6, no. 3 (August 2014): 1–29.
- Gil Tal et al., “Who Is Buying Electric Cars in California: Exploring Household and Vehicle Fleet Characteristics of New Plug-In Vehicle Owners,” Institute of Transportation Studies, University of California–Davis, February 2013.
- U.S. Bureau of the Census, American Community Survey.
- Jim Gorzelany, “Electric-Car Buyers Younger and Richer than Hybrid Owners,” Forbes, Apr. 23, 2014.
- CarMax, “2017 Hybrid and Electric Cars Survey,” July 18, 2017.
- The most common exception occurs when an electric utility provides a large industrial customer with special service or equipment used by that customer only.
- This is sometimes called a “ready-to-serve” charge.
- To some extent, time-of-use pricing can allocate costs more directly to those who cause increases in peak demand. Thus, a ZEV owner who recharges his vehicle in the early evening, when electricity demand is greatest, will pay relatively more than if he recharges the vehicle in the middle of the night, when demand is lowest.
- EnergySage, “Solar Panels and Electric Cars: Can I Use Solar as an EV Charger?” Jan. 8, 2017.
- Institute of Electrical and Electronics Engineers, “IEEE Application Guide for IEEE Std 1547, IEEE Standard for Interconnecting Distributed Resources with Electric Power Systems,” IEEE Std 1547.2-2008, Apr. 15, 2009.
- Anderson Hoke et al., “Maximum Photovoltaic Penetration Levels on Typical Distribution Feeders,” July 2012.
- EnergySage,“How Much Do Solar Panels Cost in the U.S. in 2018?” Feb. 18, 2018. According to this publication, the average household solar PV installation is 6 kW, and installation costs range from $2.71/watt to $3.57/watt. That implies a total cost between $16,260 and $21,420, before tax credits. A 10 kW system would have an installed cost between $27,100 and $35,700.
Are you interested in supporting the Manhattan Institute’s public-interest research and journalism? As a 501(c)(3) nonprofit, donations in support of MI and its scholars’ work are fully tax-deductible as provided by law (EIN #13-2912529).